Metal additive manufacturing of orthopedic bone plates: An overview
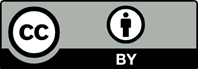
Orthopedic bone plates, traditionally made from materials such as stainless steel or titanium alloy, have been pivotal in treating fractures. However, the disparity in modulus between these metals and natural bone leads to challenges, especially stress shielding, which can hinder optimal healing and cause issues such as bone resorption. In addition, the increase in complex fractures due to osteoporosis and demographic changes also points to the limitations of standard bone plates. This evolving landscape underscores the growing need for patient-specific solutions. This review delves into the advantages and challenges concerning the material choice, design, and production processes for the additive manufacturing (AM) of bone plates. AM offers the potential to customize bone plates using detailed computerized tomography scans or topology optimization, paving the way for unparalleled customization and potentially more effective bone regeneration. However, the intricacies of AM, from choosing the right materials to final production, add layers of complexity. An innovative methodology in the field of laser-metal Additive Manufacturing, known as Material-Structure-Performance Integrated AM (MSPI-AM), is at the forefront of tackling existing challenges, with the goal of enhancing the overall process in this domain. This strategy seamlessly blends material properties, structural components, and functional performance. Enriched by the analytical capabilities of artificial intelligence, this comprehensive method aims to enhance the AM process. It envisions a future where orthopedic treatments are not just functional but also are personalized masterpieces that reflect individual patient needs and address a variety of fracture scenarios.
- Zhou J, Zhang Z, Joseph J, et al., 2021, Biomaterials and nanomedicine for bone regeneration: Progress and future prospects. Exploration, 1: 20210011. https://doi.org/10.1002/EXP.20210011
- Wildemann B, Ignatius A, Leung F, et al., 2021, Non-union bone fractures. Nat Rev Dis Primers, 7: 57. https://doi.org/10.1038/s41572-021-00289-8
- Phillips AM, 2005, Overview of the fracture healing cascade. Injury, 36: S5–S7. https://doi.org/10.1016/j.injury.2005.07.027
- Ghiasi MS, Chen J, Vaziri A, et al., 2017, Bone fracture healing in mechanobiological modeling: A review of principles and methods. Bone Rep, 6: 87–100. https://doi.org/10.1016/j.bonr.2017.03.002
- Salhotra A, Shah HN, Levi B, et al., 2020, Mechanisms of bone development and repair. Nat Rev Mol Cell Biol, 21: 696–711. https://doi.org/10.1038/s41580-020-00279-w
- Bahney CS, Zondervan RL, Allison P, et al., 2019, Cellular biology of fracture healing. J Orthop Res, 37: 35–50. https://doi.org/10.1002/jor.24170
- Li J, Qin L, Yang K, et al., 2020, Materials evolution of bone plates for internal fixation of bone fractures: A review. J Mater Sci Technol, 36: 190–208. https://doi.org/10.1016/j.jmst.2019.07.024
- Sheen JR, Mabrouk A, Garla VV, 2023, Fracture healing overview. In: StatPearls, Treasure Island, FL: StatPearls Publishing.
- Ito H, 2011, Chemokines in mesenchymal stem cell therapy for bone repair: A novel concept of recruiting mesenchymal stem cells and the possible cell sources. Mod Rheumatol, 21: 113–121. https://doi.org/10.1007/s10165-010-0357-8
- Pivonka P, Dunstan CR, 2012, Role of mathematical modeling in bone fracture healing. Bonekey Rep, 1: 221. https://doi.org/10.1038/bonekey.2012.221
- Duan ZW, Lu H, 2021, Effect of mechanical strain on cells involved in fracture healing. Orthop Surg, 13: 369–375. https://doi.org/10.1111/os.12885
- Alias MA, Buenzli PR, 2018, Osteoblasts infill irregular pores under curvature and porosity controls: A hypothesis-testing analysis of cell behaviours. Biomech Model Mechanobiol, 17: 1357–1371. https://doi.org/10.1007/s10237-018-1031-x
- Rajendran AK, Sankar D, Amirthalingam S, et al., 2023, Trends in mechanobiology guided tissue engineering and tools to study cell-substrate interactions: A brief review. Biomater Res, 27: 55. https://doi.org/10.1186/s40824-023-00393-8
- Zaheer MU, Mehboob H, Mehboob A, et al., 2022, Evaluation of the effect of bone plate modulus on the early bone healing of fractured tibia. Compos B Eng, 233: 109668. https://doi.org/10.1016/j.compositesb.2022.109668
- Kim T, See CW, Li X, et al., 2020, Orthopedic implants and devices for bone fractures and defects: Past, present and perspective. Eng Regen, 1: 6–18. https://doi.org/10.1016/j.engreg.2020.05.003
- Bartonícek J, 2010, Early history of operative treatment of fractures. Arch Orthop Trauma Surg, 130: 1385–1396. https://doi.org/10.1007/s00402-010-1082-7
- Lane WA, 1895, Some remarks on the treatment of fractures. Br Med J, 1: 861–863.
- Bagby GW, Janes JM, 1958, The effect of compression on the rate of fracture healing using a special plate. Am J Surg, 95: 761–771. https://doi.org/10.1016/0002-9610(58)90625-1
- Uhthoff HK, Poitras P, Backman DS, 2006, Internal plate fixation of fractures: Short history and recent developments. J Orthop Sci, 11: 118–126. https://doi.org/10.1007/s00776-005-0984-7
- Perren SM, 2002, Evolution of the internal fixation of long bone fractures. The scientific basis of biological internal fixation: Choosing a new balance between stability and biology. J Bone Joint Surg Br, 84: 1093–1110. https://doi.org/10.1302/0301-620x.84b8.13752
- Egol KA, Kubiak EN, Fulkerson E, et al., 2004, Biomechanics of locked plates and screws. J Orthop Trauma, 18: 488–493. https://doi.org/10.1097/00005131-200409000-00003
- Haas N, Hauke C, Schütz M, et al., 2001, Treatment of diaphyseal fractures of the forearm using the Point Contact Fixator (PC-Fix): results of 387 fractures of a prospective multicentric study (PC-Fix II). Injury, 32 Suppl 2: B51–B62. https://doi.org/10.1016/s0020-1383(01)00126-7
- Mehboob A, Chang SH, 2019, Effect of initial micro-movement of a fracture gap fastened by composite prosthesis on bone healing. Compos Struct, 226: 111213. https://doi.org/10.1016/j.compstruct.2019.111213
- Wagner M, 2003, General principles for the clinical use of the LCP. Injury, 34 Suppl 2: B31–B42. https://doi.org/10.1016/j.injury.2003.09.023
- Huiskes R, Weinans H, van Rietbergen B, 1992, The relationship between stress shielding and bone resorption around total hip stems and the effects of flexible materials. Clin Orthop Relat Res, 274: 124–134.
- Feng YJ, Lin KP, Tsai CL, et al., 2021, Influence of gap distance between bone and plate on structural stiffness and parallel interfragmental movement in far-cortical locking technique - a biomechanical study. Comput Methods Biomech Biomed Engin, 24: 1206–1211. https://doi.org/10.1080/10255842.2020.1870964
- Suwardi A, Wang F, Xue K, et al., 2022, Machine learning-driven biomaterials evolution. Adv Mater, 34: 2102703. https://doi.org/10.1002/adma.202102703
- Yang Y, He C, Dianyu E, et al., 2020, Mg bone implant: Features, developments and perspectives. Mater Des, 185: 108259. https://doi.org/10.1016/j.matdes.2019.108259
- ISO/ASTM, 2021, ISO/ASTM 52900:2021(en), Additive Manufacturing - General Principles -Fundamentals and Vocabulary. Available from: https://www.iso.org/obp/ ui/#iso: std:iso-astm:52900:ed-2:v1:en [Last accessed on 2023 Aug 07].
- Salmi M, 2021, Additive manufacturing processes in medical applications. Materials (Basel), 14: 191. https://doi.org/10.3390/ma14010191
- Vafadar A, Guzzomi F, Rassau A, et al., 2021, Advances in metal additive manufacturing: A review of common processes, industrial applications, and current challenges. Appl Sci, 11: 1213. https://doi.org/10.3390/app11031213
- Askari M, Hutchins DA, Thomas PJ, et al., 2020, Additive manufacturing of metamaterials: A review. Addit Manuf, 36: 101562. https://doi.org/10.1016/j.addma.2020.101562
- Al-Tamimi AA, Huang B, Vyas C, et al., 2019, Topology optimised metallic bone plates produced by electron beam melting: A mechanical and biological study. Int J Adv Manuf Technol, 104: 195–210. https://doi.org/10.1007/s00170-019-03866-0
- Vijayavenkataraman S, Gopinath A, Lu WF, 2020, A new design of 3D-printed orthopedic bone plates with auxetic structures to mitigate stress shielding and improve intra-operative bending. Bio Des Manuf, 3: 98–108. https://doi.org/10.1007/s42242-020-00066-8
- Kanagalingam S, Dalton C, Champneys P, et al., 2023, Detailed design for additive manufacturing and post processing of generatively designed high tibial osteotomy fixation plates. Prog Addit Manuf, 8: 409–426. https://doi.org/10.1007/s40964-022-00342-2
- Dobbe JGG, Peymani A, Roos HAL, et al., 2021, Patient-specific plate for navigation and fixation of the distal radius: A case series. Int J CARS, 16: 515–524. https://doi.org/10.1007/s11548-021-02320-5
- Teo AQA, Ng DQK, Lee P, et al., 2021, Point-of-care 3D printing: A feasibility study of using 3D printing for orthopaedic trauma. Injury, 52: 3286–3292. https://doi.org/10.1016/j.injury.2021.02.041
- Steffen C, Sellenschloh K, Willsch M, et al., 2023, Patient-specific miniplates versus patient-specific reconstruction plate: A biomechanical comparison with 3D-printed plates in mandibular reconstruction. J Mech Behav Biomed Mater, 140: 105742. https://doi.org/10.1016/j.jmbbm.2023.105742
- Nicholson J, Makaram N, Simpson A, et al., 2021, Fracture nonunion in long bones: A literature review of risk factors and surgical management. Injury, 52: S3–S11. https://doi.org/10.1016/j.injury.2020.11.029
- Roseti L, Parisi V, Petretta M, et al., 2017, Scaffolds for Bone Tissue Engineering: State of the art and new perspectives. Mater Sci Eng C Mater Biol Appl, 78: 1246–1262. https://doi.org/10.1016/j.msec.2017.05.017
- DePuy Synthes, 2023, Small Fragment Locking Compression Plate System. DePuy Synthes. J&J MedTech. Available from: https://www.jnjmedtech.com/en-US/product/small-fragment-locking-compression-plate-system [Last accessed on 2023 Jul 24].
- Millis DL, 2014, Responses of musculoskeletal tissues to disuse and remobilization. In: Levine D, editor. Canine Rehabilitation and Physical Therapy. 2nd ed. St. Louis: W.B. Saunders. p. 92–153. https://doi.org/10.1016/B978-1-4377-0309-2.00007-7
- Jahadakbar A, Nematollahi M, Safaei K, et al., 2020, Design, modeling, additive manufacturing, and polishing of stiffness-modulated porous nitinol bone fixation plates followed by thermomechanical and composition analysis. Metals, 10: 151. https://doi.org/10.3390/met10010151
- Son DS, Chang SH, 2013, The simulation of bone healing process of fractured tibia applied with composite bone plates according to the diaphyseal oblique angle and plate modulus. Compos B Eng, 45: 1325–1335. https://doi.org/10.1016/j.compositesb.2012.07.037
- Mehboob A, Chang SH, 2018, Effect of composite bone plates on callus generation and healing of fractured tibia with different screw configurations. Compos Sci Technol, 167: 96–105. https://doi.org/10.1016/j.compscitech.2018.07.039
- Al-Tamimi AA, 2021, 3D topology optimization and mesh dependency for redesigning locking compression plates aiming to reduce stress shielding. Int J Bioprint, 7: 339. https://doi.org/10.18063/ijb.v7i3.339
- Kim S, Jo Y, Choi W, et al., 2017, Biomechanical properties of 3-dimensional printed volar locking distal radius plate: Comparison with conventional volar locking plate. J Hand Surg, 42: 747.e1–747.e6. https://doi.org/10.1016/j.jhsa.2017.05.009
- Da Cruz Gomes AA, Grassi END, Da Silva PCS, et al., 2021, Mechanical behavior of a NiTi superelastic bone plate obtained by investment casting assisted by additive manufacturing. Smart Mater Struct, 30: 025009. https://doi.org/10.1088/1361-665X/abca83
- Bandyopadhyay A, Ciliveri S, Bose S, 2022, Metal additive manufacturing for load-bearing implants. J Indian Inst Sci, 102: 561–584. https://doi.org/10.1007/s41745-021-00281-x
- Ngo TD, Kashani A, Imbalzano G, et al., 2018, Additive manufacturing (3D printing): A review of materials, methods, applications and challenges. Compos B Eng, 143: 172–196. https://doi.org/10.1016/j.compositesb.2018.02.012
- Barroqueiro B, Andrade-Campos A, Valente RAF, et al., 2019, Metal additive manufacturing cycle in aerospace industry: A comprehensive review. J Manuf Mater Process, 3: 52. https://doi.org/10.3390/jmmp3030052
- Chocholata P, Kulda V, Babuska V, 2019, Fabrication of scaffolds for bone-tissue regeneration. Materials (Basel), 12: 568. https://doi.org/10.3390/ma12040568
- Hayes JS, Richards RG, 2010, The use of titanium and stainless steel in fracture fixation. Expert Rev Med Devices, 7: 843–853. https://doi.org/10.1586/erd.10.53
- Wang D, Yang Y, Han C, 2023, Additive manufacturing of metal implants and surgical plates. In: Additive Manufacturing: Materials, Functionalities and Applications. Cham: Springer International Publishing. pp. 151–203. https://doi.org/10.1007/978-3-031-04721-3_5
- Röttger A, Boes J, Theisen W, et al., 2020, Microstructure and mechanical properties of 316L austenitic stainless steel processed by different SLM devices. Int J Adv Manuf Technol, 108: 769–783. https://doi.org/10.1007/s00170-020-05371-1
- Geetha M, Singh AK, Asokamani R, et al., 2009, Ti based biomaterials, the ultimate choice for orthopaedic implants - a review. Prog Mater Sci, 54: 397–425. https://doi.org/10.1016/j.pmatsci.2008.06.004
- Liu S, Shin YC, 2019, Additive manufacturing of Ti6Al4V alloy: A review. Mater Des, 164: 107552. https://doi.org/10.1016/j.matdes.2018.107552
- Mirzaali MJ, Schwiedrzik JJ, Thaiwichai S, et al., 2016, Mechanical properties of cortical bone and their relationships with age, gender, composition and microindentation properties in the elderly. Bone, 93: 196–211. https://doi.org/10.1016/j.bone.2015.11.018
- Katzenberger MJ, Albert DL, Agnew AM, et al., 2020, Effects of sex, age, and two loading rates on the tensile material properties of human rib cortical bone. J Mech Behav Biomed Mater, 102: 103410. https://doi.org/10.1016/j.jmbbm.2019.103410
- Chen Q, Thouas GA, 2015, Metallic implant biomaterials. Mater Sci Eng R Rep, 87: 1–57. https://doi.org/10.1016/j.mser.2014.10.001
- Nadammal N, Rajput M, Gupta SK, et al., 2022, Laser powder bed fusion additive manufacturing of a low-modulus Ti-35Nb-7Zr-5Ta alloy for orthopedic applications. ACS Omega, 7: 8506–8517. https://doi.org/10.1021/acsomega.1c06261
- Li Y, Ding Y, Munir K, et al., 2019, Novel β-Ti35Zr28Nb alloy scaffolds manufactured using selective laser melting for bone implant applications. Acta Biomater, 87: 273–284. https://doi.org/10.1016/j.actbio.2019.01.051
- Batalha WC, Batalha RL, Kosiba K, et al., 2023, Effect of scanning strategy on microstructure and mechanical properties of a biocompatible Ti-35Nb-7Zr-5Ta alloy processed by laser-powder bed fusion. J Mater Res, 38: 154–164. https://doi.org/10.1557/s43578-022-00735-7
- Putra NE, Mirzaali MJ, Apachitei I, et al., 2020, Multi-material additive manufacturing technologies for Ti-, Mg-, and Fe-based biomaterials for bone substitution. Acta Biomater, 109: 1–20. https://doi.org/10.1016/j.actbio.2020.03.037
- He J, Fang J, Wei P, et al., 2021, Cancellous bone-like porous Fe@Zn scaffolds with core-shell-structured skeletons for biodegradable bone implants. Acta Biomater, 121: 665–681. https://doi.org/10.1016/j.actbio.2020.11.032
- Manam NS, Harun WSW, Shri DNA, et al., 2017, Study of corrosion in biocompatible metals for implants: A review. J Alloys Compd, 701: 698–715. https://doi.org/10.1016/j.jallcom.2017.01.196
- Kabir H, Munir K, Wen C, et al., 2021, Recent research and progress of biodegradable zinc alloys and composites for biomedical applications: Biomechanical and biocorrosion perspectives. Bioact Mater, 6: 836–879. https://doi.org/10.1016/j.bioactmat.2020.09.013
- Chaya A, Yoshizawa S, Verdelis K, et al., 2015, In vivo study of magnesium plate and screw degradation and bone fracture healing. Acta Biomater, 18: 262–269. https://doi.org/10.1016/j.actbio.2015.02.010
- Wang J, Dou J, Wang Z, et al., 2022, Research progress of biodegradable magnesium-based biomedical materials: A review. J Alloys Compd, 923: 166377. https://doi.org/10.1016/j.jallcom.2022.166377
- Bairagi D, Mandal S, 2022, A comprehensive review on biocompatible Mg-based alloys as temporary orthopaedic implants: Current status, challenges, and future prospects. J Magnes Alloys, 10: 627–669. https://doi.org/10.1016/j.jma.2021.09.005
- Hou R, Victoria-Hernandez J, Jiang P, et al., 2019, In vitro evaluation of the ZX11 magnesium alloy as potential bone plate: Degradability and mechanical integrity. Acta Biomater, 97: 608–622. https://doi.org/10.1016/j.actbio.2019.07.053
- Mo X, Zhang D, Liu K, et al., 2023, Nano-hydroxyapatite composite scaffolds loaded with bioactive factors and drugs for bone tissue engineering. Int J Mol Sci, 24: 1291. https://doi.org/10.3390/ijms24021291
- Barth T, Münch M, Seide K, et al., 2022, Additive Manufactured Versus Traditional Osteosynthesis Plates - a Finite Element Analysis. In: Conference Transactions on Additive Manufacturing Meets Medicine. p. 644. https://doi.org/10.18416/AMMM.2022.2209644
- Wu C, Zheng K, Fang J, et al., 2020, Time-dependent topology optimization of bone plates considering bone remodeling. Comput Methods Appl Mech Eng, 359: 112702. https://doi.org/10.1016/j.cma.2019.112702
- Zhang G, Li J, Zhou X, et al., 2023, The design and processing of a 3D-printed high-performance biological fixation plate. Int J Bioprint, 9: 658. https://doi.org/10.18063/ijb.v9i2.658
- Park S, Park S, Park J, et al., 2021, Design process of patient-specific osteosynthesis plates using topology optimization. J Computat Des Eng, 8: 1257–1266. https://doi.org/10.1093/jcde/qwab047
- Subasi O, Karaismailoglu B, Ashkani-Esfahani S, et al., 2023, Investigation of lattice infill parameters for additively manufactured bone fracture plates to reduce stress shielding. Comput Biol Med, 161: 107062. https://doi.org/10.1016/j.compbiomed.2023.107062
- Xu S, Ding X, Xiong M, et al., 2023, The optimal design of 3D-printed lattice bone plate by considering fracture healing mechanism. Int J Numer Methods Biomed Eng, 39: e3682. https://doi.org/10.1002/cnm.3682
- Javaid M, Haleem A, 2019, Current status and challenges of Additive manufacturing in orthopaedics: An overview. J Clin Orthop Trauma, 10: 380–386. https://doi.org/10.1016/j.jcot.2018.05.008
- Jabran A, Peach C, Zou Z, et al., 2019, Parametric design optimisation of proximal humerus plates based on finite element method. Ann Biomed Eng, 47: 601–614. https://doi.org/10.1007/s10439-018-02160-6
- Yan L, Lim JL, Lee JW, et al., 2020, Finite element analysis of bone and implant stresses for customized 3D-printed orthopaedic implants in fracture fixation. Med Biol Eng Comput, 58: 921–931. https://doi.org/10.1007/s11517-019-02104-9
- Abellán-Nebot JV, Siller HR, Vila C, et al., 2012, An experimental study of process variables in turning operations of Ti-6Al-4V and Cr-Co spherical prostheses. Int J Adv Manuf Technol, 63: 887–902. https://doi.org/10.1007/s00170-012-3955-0
- Xu M, Zhang LH, Zhang YZ, et al., 2014, Custom-made locked plating for acetabular fracture: A pilot study in 24 consecutive cases. Orthopedics, 37: e660–e670. https://doi.org/10.3928/01477447-20140626-59
- Xie P, Ouyang H, Deng Y, et al., 2017, Comparison of conventional reconstruction plate versus direct metal laser sintering plate: An in vitro mechanical characteristics study. J Orthop Surg Res, 12: 128. https://doi.org/10.1186/s13018-017-0628-6
- Ballard DH, Mills P, Duszak R Jr., et al., 2020, Medical 3D printing cost-savings in orthopedic and maxillofacial surgery: Cost analysis of operating room time saved with 3D printed anatomic models and surgical guides. Acad Radiol, 27: 1103–1113. https://doi.org/10.1016/j.acra.2019.08.011
- Davis R, Singh A, Jackson MJ, et al., 2022, A comprehensive review on metallic implant biomaterials and their subtractive manufacturing. Int J Adv Manuf Technol, 120: 1473–1530. https://doi.org/10.1007/s00170-022-08770-8
- Stepanovska J, Matejka R, Otahal M, et al., 2020, The effect of various surface treatments of Ti6Al4V on the growth and osteogenic differentiation of adipose tissue-derived stem cells. Coatings, 10: 762. https://doi.org/10.3390/coatings10080762
- Jia Z, Xu X, Zhu D, et al., 2023, Design, printing, and engineering of regenerative biomaterials for personalized bone healthcare. Prog Mater Sci, 134: 101072. https://doi.org/10.1016/j.pmatsci.2023.101072
- Lu H, Wu L, Wei H, et al., 2022, Microstructural evolution and tensile property enhancement of remanufactured Ti6Al4V using hybrid manufacturing of laser directed energy deposition with laser shock peening. Addit Manuf, 55: 102877. https://doi.org/10.1016/j.addma.2022.102877
- Lalegani Dezaki M, Serjouei A, Zolfagharian A, et al., 2022, A review on additive/subtractive hybrid manufacturing of directed energy deposition (DED) process. Adv Powder Mater, 1: 100054. https://doi.org/10.1016/j.apmate.2022.100054
- Shalabi MM, Gortemaker A, Hof MAV, et al., 2006, Implant surface roughness and bone healing: A systematic review. J Dent Res, 85: 496–500. https://doi.org/10.1177/154405910608500603
- Rønold HJ, Lyngstadaas SP, Ellingsen JE, 2003, Analysing the optimal value for titanium implant roughness in bone attachment using a tensile test. Biomaterials, 24: 4559–4564. https://doi.org/10.1016/S0142-9612(03)00256-4
- Gupta SK, Shahidsha N, Bahl S, et al., 2021, Enhanced biomechanical performance of additively manufactured Ti-6Al-4V bone plates. J Mech Behav Biomed Mater, 119: 104552. https://doi.org/10.1016/j.jmbbm.2021.104552
- Gittens RA, McLachlan T, Olivares-Navarrete R, et al., 2011, The effects of combined micron-/submicron-scale surface roughness and nanoscale features on cell proliferation and differentiation. Biomaterials, 32: 3395–3403. https://doi.org/10.1016/j.biomaterials.2011.01.029
- Haleem A, Javaid M, 2020, 3D printed medical parts with different materials using additive manufacturing. Clin Epidemiol Glob Health, 8: 215–223. https://doi.org/10.1016/j.cegh.2019.08.002
- Omori S, Murase T, Kataoka T, et al., 2014, Three-dimensional corrective osteotomy using a patient-specific osteotomy guide and bone plate based on a computer simulation system: Accuracy analysis in a cadaver study. Int J Med Robot, 10: 196–202. https://doi.org/10.1002/rcs.1530
- Chung CY, 2018, A simplified application (APP) for the parametric design of screw-plate fixation of bone fractures. J Mech Behav Biomed Mater, 77: 642–648. https://doi.org/10.1016/j.jmbbm.2017.10.025
- Mohandes Y, Tahani M, Rouhi G, et al., 2021, A mechanobiological approach to find the optimal thickness for the locking compression plate: Finite element investigations. Proc Inst Mech Eng H, 235: 408–418. https://doi.org/10.1177/0954411920985757
- Schader JF, Mischler D, Dauwe J, et al., 2022, One size may not fit all: Patient-specific computational optimization of locking plates for improved proximal humerus fracture fixation. J Shoulder Elbow Surg, 31: 192–200. https://doi.org/10.1016/j.jse.2021.06.012
- Gu D, Shi X, Poprawe R, et al., 2021, Material-structure-performance integrated laser-metal additive manufacturing. Science, 372: eabg1487. https://doi.org/10.1126/science.abg1487
- Lima DD, Mantri SA, Mikler CV, et al., 2017, Laser additive processing of a functionally graded internal fracture fixation plate. Mater Des, 130: 8–15. https://doi.org/10.1016/j.matdes.2017.05.034
- Babu SS, Mourad AHI, Harib KH, et al., 2023, Recent developments in the application of machine-learning towards accelerated predictive multiscale design and additive manufacturing. Virtual Phys Prototyp, 18: e2141653. https://doi.org/10.1080/17452759.2022.2141653
- Caiazzo F, Caggiano A, 2018, Laser direct metal deposition of 2024 Al Alloy: Trace geometry prediction via machine learning. Materials (Basel), 11: 444. https://doi.org/10.3390/ma11030444
- Le C, Kolasangiani K, Nayyeri P, et al., 2023, Experimental and numerical investigation of 3D-Printed bone plates under four-point bending load utilizing machine learning techniques. J Mech Behav Biomed Mater, 143: 105885. https://doi.org/10.1016/j.jmbbm.2023.105885
- Wang C, Tan XP, Tor SB, et al., 2020, Machine learning in additive manufacturing: State-of-the-art and perspectives. Addit Manuf, 36: 101538. https://doi.org/10.1016/j.addma.2020.101538
- Einhorn TA, Gerstenfeld LC, 2015, Fracture healing: Mechanisms and interventions. Nat Rev Rheumatol, 11: 45–54. https://doi.org/10.1038/nrrheum.2014.164
- Ahn DG, 2021, Directed energy deposition (DED) process: State of the art. Int J Precis Eng Manuf Green Tech, 8: 703–742. https://doi.org/10.1007/s40684-020-00302-7
- Singh A, Kapil S, Das M, 2020, A comprehensive review of the methods and mechanisms for powder feedstock handling in directed energy deposition. Addit Manuf, 35: 101388. https://doi.org/10.1016/j.addma.2020.101388
- Casalino G, Karamimoghadam M, Contuzzi N, 2023, Metal wire additive manufacturing: A comparison between arc laser and laser/arc heat sources. Inventions, 8: 52. https://doi.org/10.3390/inventions8020052
- Bassis M, Ron T, Leon A, et al., 2022, The influence of intralayer porosity and phase transition on corrosion fatigue of additively manufactured 316L stainless steel obtained by direct energy deposition process. Materials, 15: 5481. https://doi.org/10.3390/ma15165481
- Bambach M, Sizova I, Kies F, et al., 2021, Directed energy deposition of Inconel 718 powder, cold and hot wire using a six-beam direct diode laser set-up. Addit Manuf, 47: 102269. https://doi.org/10.1016/j.addma.2021.102269
- Negi S, Nambolan AA, Kapil S, et al., 2020, Review on electron beam based additive manufacturing. Rapid Prototyp J, 26: 485–498.
- Tilton M, Lewis GS, Bok Wee H, et al., 2020, Additive manufacturing of fracture fixation implants: Design, material characterization, biomechanical modeling and experimentation. Addit Manuf, 33: 101137. https://doi.org/10.1016/j.addma.2020.101137
- Schappo H, Giry K, Salmoria G, et al., 2023, Polymer/ calcium phosphate biocomposites manufactured by selective laser sintering: An overview. Prog Addit Manuf, 8: 285–301. https://doi.org/10.1007/s40964-022-00332-4