Toward better drug development: Three-dimensional bioprinting in toxicological research
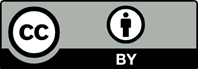
The importance of three-dimensional (3D) models in pharmacological tests and personalized therapies is significant. These models allow us to gain insight into the cell response during drug absorption, distribution, metabolism, and elimination in an organlike system and are suitable for toxicological testing. In personalized and regenerative medicine, the precise characterization of artificial tissues or drug metabolism processes is more than crucial to gain the safest and the most effective treatment for the patients. Using these 3D cell cultures derived directly from patient, such as spheroids, organoids, and bioprinted structures, allows for testing drugs before administration to the patient. These methods allow us to select the most appropriate drug for the patient. Moreover, they provide chance for better recovery of patients, since time is not wasted during therapy switching. These models could be used in applied and basic research as well, because their response to treatments is quite similar to that of the native tissue. Furthermore, they may replace animal models in the future because these methods are cheaper and can avoid interspecies differences. This review puts a spotlight on this dynamically evolving area and its application in toxicological testing.
1. Li AP, 2001, Screening for human ADME/Tox drug properties in drug discovery. Drug Discov Today, 6: 357–366. https://doi.org/10.1016/s1359-6446(01)01712-3
2. Wishart DS, 2016, Emerging applications of metabolomics in drug discovery and precision medicine. Nat Rev Drug Discov, 15: 473–484. https://doi.org/10.1038/nrd.2016.32
3. Hingorani AD, Kuan V, Finan C, et al., 2019, Improving the odds of drug development success through human genomics: Modelling study. Sci Rep, 9: 18911. https://doi.org/10.1038/s41598-019-54849-w
4. Serras AS, Rodrigues JS, Cipriano M, et al., 2021, A critical perspective on 3D liver models for drug metabolism and toxicology studies. Front Cell Dev Biol, 9: 626805. https://doi.org/10.3389/fcell.2021.626805
5. Park Y, Huh KM, Kang SW, 2021, Applications of biomaterials in 3D cell culture and contributions of 3D cell culture to drug development and basic biomedical research. Int J Mol Sci, 22: 2491. https://doi.org/10.3390/ijms22052491
6. Ma C, Peng Y, Li H, et al., 2021, Organ-on-a-chip: A new paradigm for drug development. Trends Pharmacol Sci, 42: 119–133. https://doi.org/10.1016/j.tips.2020.11.009
7. Saltsman JA, Hammond WJ, Narayan NJ, et al., 2020, A human organoid model of aggressive hepatoblastoma for disease modeling and drug testing. Cancers (Basel), 12: 2668. https://doi.org/10.3390/cancers12092668
8. Takahashi T, 2019, Organoids for drug discovery and personalized medicine. Annu Rev Pharmacol Toxicol, 59: 447–462. https://doi.org/10.1146/annurev-pharmtox-010818-021108
9. Devarasetty M, Mazzocchi AR, Skardal A, 2018, Applications of bioengineered 3D tissue and tumor organoids in drug development and precision medicine: Current and future. BioDrugs, 32: 53–68. https://doi.org/10.1007/s40259-017-0258-x
10. Peng W, Datta P, Ayan B, et al., 2017, 3D bioprinting for drug discovery and development in pharmaceutics. Acta Biomater, 57: 26–46. https://doi.org/10.1016/j.actbio.2017.05.025
11. Sun D, Gao W, Hu H, et al., 2022, Why 90% of clinical drug development fails and how to improve it? Acta Pharm Sin B, 12: 3049–3062. https://doi.org/10.1016/j.apsb.2022.02.002
12. Smith DA, Beaumont K, Maurer TS, et al., 2019, Clearance in drug design. J Med Chem, 62: 2245–2255. https://doi.org/10.1021/acs.jmedchem.8b01263
13. Phua J, Lee KH, 2008, Liver support devices. Curr Opin Crit Care, 14: 208–215. https://doi.org/10.1097/MCC.0b013e3282f70057
14. Zhang X, Jiang T, Chen D, et al., 2020, Three-dimensional liver models: State of the art and their application for hepatotoxicity evaluation. Crit Rev Toxicol, 50: 279–309. https://doi.org/10.1080/10408444.2020.1756219
15. Roden M, 2016, The liver in focus. Diabetologia, 59: 1095–1097. https://doi.org/10.1007/s00125-016-3911-x
16. Lorente S, Hautefeuille M, Sanchez-Cedillo A, 2020, The liver, a functionalized vascular structure. Sci Rep, 10: 16194. https://doi.org/10.1038/s41598-020-73208-8
17. Shin D, Monga SP, 2013, Cellular and molecular basis of liver development. Compr Physiol, 3(2): 799–815. https://doi.org/10.1002/cphy.c120022
18. Stockert RJ, Wolkoff AW, 2001, Cellular and molecular biology of the liver. Curr Opin Gastroenterol, 17: 205–210. https://doi.org/10.1097/00001574-200105000-00003
19. Popper H, 1956, Correlation of liver structure and function. Q Bull Northwest Univ Med Sch, 30: 325–330. http://www.ncbi.nlm.nih.gov/pubmed/13408428
20. Michalopoulos GK, Bhushan B, 2021, Liver regeneration: biological and pathological mechanisms and implications. Nat Rev Gastroenterol Hepatol, 18: 40–55. https://doi.org/10.1038/s41575-020-0342-4
21. Poyck PP, Hoekstra R, Chhatta A, et al., 2007, Time-related analysis of metabolic liver functions, cellular morphology, and gene expression of hepatocytes cultured in the bioartificial liver of the academic medical center in Amsterdam (AMC-BAL). Tissue Eng, 13: 1235–1246. https://doi.org/10.1089/ten.2006.0343
22. Pahlavan PS, Feldmann RE Jr., Zavos C, et al., 2006, Prometheus’ challenge: molecular, cellular and systemic aspects of liver regeneration. J Surg Res, 134: 238–251. https://doi.org/10.1016/j.jss.2005.12.011
23. Vellonen KS, Malinen M, Mannermaa E, et al., 2014, A critical assessment of in vitro tissue models for ADME and drug delivery. J Control Release, 190: 94–114. https://doi.org/10.1016/j.jconrel.2014.06.044
24. Madden LR, Nguyen TV, Garcia-Mojica S, et al., 2018, Bioprinted 3D primary human intestinal tissues model aspects of native physiology and ADME/Tox functions. iScience, 2: 156–167. https://doi.org/10.1016/j.isci.2018.03.015
25. Faber KN, Muller M, Jansen PL, 2003, Drug transport proteins in the liver. Adv Drug Deliv Rev, 55: 107–124. https://doi.org/10.1016/s0169-409x(02)00173-4
26. Sawant-Basak A, Obach RS, 2018, Emerging models of drug metabolism, transporters, and toxicity. Drug Metab Dispos, 46: 1556–1561. https://doi.org/10.1124/dmd.118.084293
27. Jetter A, Kullak-Ublick GA, 2020, Drugs and hepatic transporters: A review. Pharmacol Res, 154: 104234. https://doi.org/10.1016/j.phrs.2019.04.018
28. Gholam PM, 2020, A focus on drug-induced liver injury. Clin Liver Dis, 24: xiii–xiv. https://doi.org/10.1016/j.cld.2019.10.001
29. Beckwitt CH, Clark AM, Wheeler S, et al., 2018, Liver “organ on a chip”. Exp Cell Res, 363: 15–25. https://doi.org/10.1016/j.yexcr.2017.12.023
30. Shimoda H, Yagi H, Higashi H, et al., 2019, Decellularized liver scaffolds promote liver regeneration after partial hepatectomy. Sci Rep, 9: 12543. https://doi.org/10.1038/s41598-019-48948-x
31. Chen Y, Geerts S, Jaramillo M, et al., 2018, Preparation of decellularized liver scaffolds and recellularized liver grafts. Methods Mol Biol, 1577: 255–270. https://doi.org/10.1007/7651_2017_56
32. Vatakuti S, Olinga P, Pennings JL, et al., 2017, Validation of precision-cut liver slices to study drug-induced cholestasis: A transcriptomics approach. Arch Toxicol, 91: 1401–1412. https://doi.org/10.1007/s00204-016-1778-8
33. Othman A, Ehnert S, Dropmann A, et al., 2020, Precision-cut liver slices as an alternative method for long-term hepatotoxicity studies. Arch Toxicol, 94: 2889–2891. https://doi.org/10.1007/s00204-020-02861-9
34. Steimberg N, Bertero A, Chiono V, et al., 2020, iPS, organoids and 3D models as advanced tools for in vitro toxicology. ALTEX, 37: 136–140. https://doi.org/10.14573/altex.1911071
35. Torok G, Erdei Z, Lilienberg J, et al., 2020, The importance of transporters and cell polarization for the evaluation of human stem cell-derived hepatic cells. PLoS One, 15: e0227751. https://doi.org/10.1371/journal.pone.0227751
36. Nguyen DG, Funk J, Robbins JB, et al., 2016, Bioprinted 3D primary liver tissues allow assessment of organ-level response to clinical drug induced toxicity in vitro. PLoS One, 11: e0158674. https://doi.org/10.1371/journal.pone.0158674
37. Bell CC, Hendriks DF, Moro SM, et al., 2016, Characterization of primary human hepatocyte spheroids as a model system for drug-induced liver injury, liver function and disease. Sci Rep, 6: 25187. https://doi.org/10.1038/srep25187
38. Kanebratt KP, Janefeldt A, Vilen L, et al., 2021, Primary human hepatocyte spheroid model as a 3D in vitro platform for metabolism studies. J Pharm Sci, 110: 422–431. https://doi.org/10.1016/j.xphs.2020.10.043
39. Li F, Cao L, Parikh S, et al., 2020, Three-dimensional spheroids with primary human liver cells and differential roles of kupffer cells in drug-induced liver injury. J Pharm Sci, 109: 1912–1923. https://doi.org/10.1016/j.xphs.2020.02.021
40. Ware BR, Liu JS, Monckton CP, et al., 2021, Micropatterned coculture with 3T3-J2 fibroblasts enhances hepatic functions and drug screening utility of heparg cells. Toxicol Sci, 181: 90–104. https://doi.org/10.1093/toxsci/kfab018
41. Trask OJ Jr., Moore A, LeCluyse EL, 2014, A micropatterned hepatocyte coculture model for assessment of liver toxicity using high-content imaging analysis. Assay Drug Dev Technol, 12: 16–27. https://doi.org/10.1089/adt.2013.525
42. Liu Y, Li H, Yan S, et al., 2014, Hepatocyte cocultures with endothelial cells and fibroblasts on micropatterned fibrous mats to promote liver-specific functions and capillary formation capabilities. Biomacromolecules, 15: 1044–1054. https://doi.org/10.1021/bm401926k
43. Khetani SR, Kanchagar C, Ukairo O, et al., 2013, Use of micropatterned cocultures to detect compounds that cause drug-induced liver injury in humans. Toxicol Sci, 132: 107–117. https://doi.org/10.1093/toxsci/kfs326
44. Choi YJ, Kim H, Kim JW, et al., 2018, Hepatic esterase activity is increased in hepatocyte-like cells derived from human embryonic stem cells using a 3D culture system. Biotechnol Lett, 40: 755–763. https://doi.org/10.1007/s10529-018-2528-1
45. Sharma VR, Shrivastava A, Gallet B, et al., 2019, Canalicular domain structure and function in matrix-free hepatic spheroids. Biomater Sci, 8: 485–496. https://doi.org/10.1039/c9bm01143a
46. Elje E, Mariussen E, Moriones OH, et al., 2020, Hepato(Geno) toxicity assessment of nanoparticles in a HepG2 liver spheroid model. Nanomaterials (Basel), 10: 545. https://doi.org/10.3390/nano10030545
47. Flores-Torres S, Peza-Chavez O, Kuasne H, et al., 2021, Alginate-gelatin-matrigel hydrogels enable the development and multigenerational passaging of patient-derived 3D bioprinted cancer spheroid models. Biofabrication 13: 025001. https://doi.org/10.1088/1758-5090/abdb87
48. Gunti S, Hoke AT, Vu KP, et al., 2021, Organoid and spheroid tumor models: Techniques and applications. Cancers (Basel), 13: 874. https://doi.org/10.3390/cancers13040874
49. Kim SJ, Kim EM, Yamamoto M, et al., 2020, Engineering multi-cellular spheroids for tissue engineering and regenerative medicine. Adv Healthc Mater, 9: e2000608. https://doi.org/10.1002/adhm.202000608
50. Proctor WR, Foster AJ, Vogt J, et al., 2017, Utility of spherical human liver microtissues for prediction of clinical drug-induced liver injury. Arch Toxicol, 91: 2849–2863. https://doi.org/10.1007/s00204-017-2002-1
51. Wang Z, Luo X, Anene-Nzelu C, et al., 2015, HepaRG culture in tethered spheroids as an in vitro three-dimensional model for drug safety screening. J Appl Toxicol, 35: 909–917. https://doi.org/10.1002/jat.3090
52. Hendriks DF, Vorrink SU, Smutny T, et al., 2020, Clinically relevant cytochrome P450 3A4 induction mechanisms and drug screening in three-dimensional spheroid cultures of primary human hepatocytes. Clin Pharmacol Ther, 108: 844–855. https://doi.org/10.1002/cpt.1860
53. Ingelman-Sundberg M, Lauschke VM, 2021, 3D human liver spheroids for translational pharmacology and toxicology. Basic Clin Pharmacol Toxicol, 130: 5–15. https://doi.org/10.1111/bcpt.13587
54. Sant S, Johnston PA, 2017, The production of 3D tumor spheroids for cancer drug discovery. Drug Discov Today Technol, 23: 27–36. https://doi.org/10.1016/j.ddtec.2017.03.002
55. Hurrell T, Ellero AA, Masso ZF, et al., 2018, Characterization and reproducibility of HepG2 hanging drop spheroids toxicology in vitro. Toxicol In Vitro, 50: 86–94. https://doi.org/10.1016/j.tiv.2018.02.013
56. Pinto B, Henriques AC, Silva PMA, et al., 2020, Three-dimensional spheroids as in vitro preclinical models for cancer research. Pharmaceutics, 12: 1186. https://doi.org/10.3390/pharmaceutics12121186
57. Penzes A, Abdelwahab EM, Rapp J, et al., 2017, Toxicology studies of primycin-sulphate using a three-dimensional (3D) in vitro human liver aggregate model. Toxicol Lett, 281: 44–52. https://doi.org/10.1016/j.toxlet.2017.09.005
58. Lancaster MA, Knoblich JA, 2014, Organogenesis in a dish: Modeling development and disease using organoid technologies. Science, 345: 1247125. https://doi.org/10.1126/science.1247125
59. Simian M, Bissell MJ, 2017, Organoids: A historical perspective of thinking in three dimensions. J Cell Biol, 216: 31–40. https://doi.org/10.1083/jcb.201610056
60. Fatehullah A, Tan SH, Barker N, 2016, Organoids as an in vitro model of human development and disease. Nat Cell Biol, 18: 246–254. https://doi.org/10.1038/ncb3312
61. Skottvoll FS, Hansen FA, Harrison S, et al., 2021, Electromembrane extraction and mass spectrometry for liver organoid drug metabolism studies. Anal Chem, 93: 3576–3585. https://doi.org/10.1021/acs.analchem.0c05082
62. Nuciforo S, Heim MH, 2021, Organoids to model liver disease. JHEP Rep, 3: 100198. https://doi.org/10.1016/j.jhepr.2020.100198
63. Zhu M, Huang Y, Bian S, et al., 2020, Organoid: Current implications and pharmaceutical applications in liver diseases. Curr Mol Pharmacol, 14: 498–508. https://doi.org/10.2174/1874467213666201217115854
64. Messina A, Luce E, Hussein M, et al., 2020, Pluripotent-stem-cell-derived hepatic cells: Hepatocytes and organoids for liver therapy and regeneration. Cells, 9: 420. https://doi.org/10.3390/cells9020420
65. Kim J, Koo BK, Knoblich JA, 2020, Human organoids: model systems for human biology and medicine. Nat Rev Mol Cell Biol, 21: 571–584. https://doi.org/10.1038/s41580-020-0259-3
66. Driehuis E, Kretzschmar K, Clevers H, 2020, Establishment of patient-derived cancer organoids for drug-screening applications. Nat Protoc, 15: 3380–3409. https://doi.org/10.1038/s41596-020-0379-4
67. Corro C, Novellasdemunt L, Li VS, 2020, A brief history of organoids. Am J Physiol Cell Physiol, 319: C151–C165. https://doi.org/10.1152/ajpcell.00120.2020
68. Prior N, Inacio P, Huch M, 2019, Liver organoids: from basic research to therapeutic applications. Gut, 68: 2228–2237. https://doi.org/10.1136/gutjnl-2019-319256
69. Mun SJ, Ryu JS, Lee MO, et al., 2019, Generation of expandable human pluripotent stem cell-derived hepatocyte-like liver organoids. J Hepatol, 71: 970–985. https://doi.org/10.1016/j.jhep.2019.06.030
70. Augustyniak J, Bertero A, Coccini T, et al., 2019, Organoids are promising tools for species-specific in vitro toxicological studies. J Appl Toxicol, 39: 1610–1622. https://doi.org/10.1002/jat.3815
71. Akbari S, Arslan N, Senturk S, et al., 2019, Next-generation liver medicine using organoid models. Front Cell Dev Biol, 7: 345. https://doi.org/10.3389/fcell.2019.00345
72. Nuciforo S, Fofana I, Matter MS, et al., 2018, Organoid models of human liver cancers derived from tumor needle biopsies. Cell Rep, 24: 1363–1376. https://doi.org/10.1016/j.celrep.2018.07.001
73. Hu H, Gehart H, Artegiani B, et al., 2018, Long-term expansion of functional mouse and human hepatocytes as 3D organoids. Cell, 175: 1591–1606 e1519. https://doi.org/10.1016/j.cell.2018.11.013
74. Artegiani B, Clevers H, 2018, Use and application of 3D-organoid technology. Hum Mol Genet, 27: R99–R107. https://doi.org/10.1093/hmg/ddy187
75. Schneeberger K, Spee B, Costa P, et al., 2017, Converging biofabrication and organoid technologies: the next frontier in hepatic and intestinal tissue engineering? Biofabrication, 9: 013001. https://doi.org/10.1088/1758-5090/aa6121
76. Dutta D, Heo I, Clevers H, 2017, Disease modeling in stem cell-derived 3D organoid systems. Trends Mol Med, 23: 393–410. https://doi.org/10.1016/j.molmed.2017.02.007
77. Leite SB, Roosens T, El Taghdouini A, et al., 2016, Novel human hepatic organoid model enables testing of drug-induced liver fibrosis in vitro. Biomaterials, 78: 1–10. https://doi.org/10.1016/j.biomaterials.2015.11.026
78. Gilazieva Z, Ponomarev A, Rutland C, et al., 2020, Promising applications of tumor spheroids and organoids for personalized medicine. Cancers (Basel), 12: 2727. https://doi.org/10.3390/cancers12102727
79. Chen EP, Toksoy Z, Davis BA, et al., 2021, 3D Bioprinting of vascularized tissues for in vitro and in vivo applications. Front Bioeng Biotechnol, 9: 664188. https://doi.org/10.3389/fbioe.2021.664188
80. Lee JM, Sing SL, Zhou M, et al., 2018, 3D bioprinting processes: A perspective on classification and terminology. Int J Bioprint, 4: 151. https://doi.org/10.18063/IJB.v4i2.151
81. Hagenbuchner J, Nothdurfter D, Ausserlechner MJ, 2021, 3D bioprinting: Novel approaches for engineering complex human tissue equivalents and drug testing. Essays Biochem, 65: 417–427. https://doi.org/10.1042/EBC20200153
82. Nguyen DG, Pentoney SL Jr., 2017, Bioprinted three dimensional human tissues for toxicology and disease modeling. Drug Discov Today Technol, 23: 37–44. https://doi.org/10.1016/j.ddtec.2017.03.001
83. Kryou C, Leva V, Chatzipetrou M, et al., 2019, Bioprinting for liver transplantation. Bioengineering (Basel), 6: 95. https://doi.org/10.3390/bioengineering6040095
84. Tasoglu S, Demirci U, 2013, Bioprinting for stem cell research. Trends Biotechnol, 31: 10–19. https://doi.org/10.1016/j.tibtech.2012.10.005
85. Agarwal T, Banerjee D, Konwarh R, et al., 2021, Recent advances in bioprinting technologies for engineering hepatic tissue. Mater Sci Eng C Mater Biol Appl, 123: 112013. https://doi.org/10.1016/j.msec.2021.112013
86. Vurat MT, Ergun C, Elcin AE, et al., 2020, 3D bioprinting of tissue models with customized bioinks. Adv Exp Med Biol, 1249: 67–84. https://doi.org/10.1007/978-981-15-3258-0_5
87. Tandon R, Froghi S, 2020, Artificial liver support systems. J Gastroenterol Hepatol, 36: 1164–1179. https://doi.org/10.1111/jgh.15255
88. Matai I, Kaur G, Seyedsalehi A, et al., 2020, Progress in 3D bioprinting technology for tissue/organ regenerative engineering. Biomaterials, 226: 119536. https://doi.org/10.1016/j.biomaterials.2019.119536
89. Ma L, Wu Y, Li Y, et al., 2020, Current advances on 3D-bioprinted liver tissue models. Adv Healthc Mater, 9: e2001517. https://doi.org/10.1002/adhm.202001517
90. Wang X, 2019, Advanced polymers for three-dimensional (3D) organ bioprinting. Micromachines (Basel), 10: 814. https://doi.org/10.3390/mi10120814
91. He YT, Qi YN, Zhang BQ, et al., 2019, Bioartificial liver support systems for acute liver failure: A systematic review and meta-analysis of the clinical and preclinical literature. World J Gastroenterol, 25: 3634–3648. https://doi.org/10.3748/wjg.v25.i27.3634
92. Chamuleau RA, 2009, Future of bioartificial liver support. World J Gastrointest Surg, 1: 21–25. https://doi.org/10.4240/wjgs.v1.i1.21
93. Lelievre SA, Kwok T, Chittiboyina S, 2017, Architecture in 3D cell culture: An essential feature for in vitro toxicology. Toxicol In Vitro, 45: 287–295. https://doi.org/10.1016/j.tiv.2017.03.012
94. Hong N, Yang GH, Lee J, et al., 2018, 3D bioprinting and its in vivo applications. J Biomed Mater Res B Appl Biomater, 106: 444–459. https://doi.org/10.1002/jbm.b.33826
95. Yu J, Park SA, Kim WD, et al., 2020, Current advances in 3D bioprinting technology and its applications for tissue engineering. Polymers (Basel), 12: 2958. https://doi.org/10.3390/polym12122958
96. Vernerey FJ, Sridhar SL, Muralidharan A, et al., 2021, Mechanics of 3D cell-hydrogel interactions: Experiments, models, and mechanisms. Chem Rev, 121: 11085–11148. https://doi.org/10.1021/acs.chemrev.1c00046
97. Hauser PV, Chang HM, Nishikawa M, et al., 2021, Bioprinting scaffolds for vascular tissues and tissue vascularization. Bioengineering (Basel), 8: 178. https://doi.org/10.3390/bioengineering8110178
98. Panwar A, Tan LP, 2016, Current status of bioinks for micro-extrusion-based 3D bioprinting. Molecules, 21: 685. https://doi.org/10.3390/molecules21060685
99. Zhuang P, Ng WL, An J, et al., 2019, Layer-by-layer ultraviolet assisted extrusion-based (UAE) bioprinting of hydrogel constructs with high aspect ratio for soft tissue engineering applications. PLoS One, 14: e0216776. https://doi.org/10.1371/journal.pone.0216776
100. Li X, Liu B, Pei B, et al., 2020, Inkjet bioprinting of biomaterials. Chem Rev, 120: 10793–10833. https://doi.org/10.1021/acs.chemrev.0c00008
101. Gudapati H, Dey M, Ozbolat I, 2016, A comprehensive review on droplet-based bioprinting: Past, present and future. Biomaterials, 102: 20–42. https://doi.org/10.1016/j.biomaterials.2016.06.012
102. Mandrycky C, Wang Z, Kim K, et al., 2016, 3D bioprinting for engineering complex tissues. Biotechnol Adv, 34: 422–434. https://doi.org/10.1016/j.biotechadv.2015.12.011
103. Xiang Y, Miller K, Guan J, et al., 2022, 3D bioprinting of complex tissues in vitro: State-of-the-art and future perspectives. Arch Toxicol, 96: 691–710. https://doi.org/10.1007/s00204-021-03212-y
104. Ma X, Liu J, Zhu W, et al., 2018, 3D bioprinting of functional tissue models for personalized drug screening and in vitro disease modeling. Adv Drug Deliv Rev, 132: 235–251. https://doi.org/10.1016/j.addr.2018.06.011
105. Pati F, Gantelius J, Svahn HA, 2016, 3D bioprinting of tissue/ organ models. Angew Chem Int Ed Engl, 55: 4650–4665. https://doi.org/10.1002/anie.201505062
106. Ng WL, Huang X, Shkolnikov V, et al., 2022, Controlling droplet impact velocity and droplet volume: Key factors to achieving high cell viability in sub-nanoliter droplet-based bioprinting. Int J Bioprint, 8: 424. https://doi.org/10.18063/ijb.v8i1.424
107. Kacarevic ZP, Rider PM, Alkildani S, et al., 2018, An introduction to 3D bioprinting: Possibilities, challenges and future aspects. Materials (Basel), 11: 2199. https://doi.org/10.3390/ma11112199
108. Gao D, Zhou JG, 2019, Designs and applications of electrohydrodynamic 3D printing. Int J Bioprint, 5: 172. https://doi.org/10.18063/ijb.v5i1.172
109. Ng WL, Lee JM, Yeong WY, et al., 2017, Microvalve-based bioprinting-process, bio-inks and applications. Biomater Sci, 5: 632–647. https://doi.org/10.1039/c6bm00861e
110. Choi YJ, Park H, Ha DHi et al., 2021, 3D bioprinting of in vitro models using hydrogel-based bioinks. Polymers (Basel), 13: 366. https://doi.org/10.3390/polym13030366
111. Li W, Mille LS, Robledo JA, et al., 2020, Recent advances in formulating and processing biomaterial inks for vat polymerization-based 3D printing. Adv Healthc Mater, 9: e2000156. https://doi.org/10.1002/adhm.202000156
112. Ng WL, Lee JM, Zhou M, et al., 2020, Vat polymerization-based bioprinting-process, materials, applications and regulatory challenges. Biofabrication, 12: 022001. https://doi.org/10.1088/1758-5090/ab6034
113. Jing X, Fu H, Yu B, et al., 2022, Two-photon polymerization for 3D biomedical scaffolds: Overview and updates. Front Bioeng Biotechnol, 10: 994355. https://doi.org/10.3389/fbioe.2022.994355
114. Huang Z, Tsui G, Deng Y, et al., 2020, Two-photon polymerization nanolithography technology for fabrication of stimulus-responsive micro/nano-structures for biomedical applications. Nanotechnol Rev, 9: 1118–1136. https://doi.org/10.1515/ntrev-2020-0073
115. Otuka AJ, Tomazio NB, Paula KT, et al., 2021, Two-photon polymerization: functionalized microstructures, micro-resonators, and bio-scaffolds. Polymers (Basel), 13: 1994. https://doi.org/10.3390/polym13121994
116. Fransen MF, Addario G, Bouten CV, et al., 2021, Bioprinting of kidney in vitro models: Cells, biomaterials, and manufacturing techniques. Essays Biochem, 65: 587–602. https://doi.org/10.1042/EBC20200158
117. Radi ZA, 2019, Kidney pathophysiology, toxicology, and
drug-induced injury in drug development. Int J Toxicol, 38: 215–227. https://doi.org/10.1177/1091581819831701
118. Pregosin NC, Bronstein R, Mallipattu SK, 2021, Recent advances in kidney bioengineering. Front Pediatr, 9: 743301. https://doi.org/10.3389/fped.2021.743301
119. Homan KA, Kolesky DB, Skylar-Scott MA, et al., 2016, Bioprinting of 3D convoluted renal proximal tubules on perfusable chips. Sci Rep, 6: 34845. https://doi.org/10.1038/srep34845
120. Lin NY, Homan KA, Robinson SS, et al., 2019, Renal reabsorption in 3D vascularized proximal tubule models. Proc Natl Acad Sci U S A, 116: 5399–5404. https://doi.org/10.1073/pnas.1815208116
121. Lawlor KT, Vanslambrouck JM, Higgins JW, et al., 2021, Cellular extrusion bioprinting improves kidney organoid reproducibility and conformation. Nat Mater, 20: 260–271. https://doi.org/10.1038/s41563-020-00853-9
122. King SM, Higgins JW, Nino CR, et al., 2017, 3D proximal tubule tissues recapitulate key aspects of renal physiology to enable nephrotoxicity testing. Front Physiol, 8: 123. https://doi.org/10.3389/fphys.2017.00123
123. Gao C, Lu C, Jian Z, et al., 2021, 3D bioprinting for fabricating artificial skin tissue. Colloids Surf B Biointerfaces, 208: 112041. https://doi.org/10.1016/j.colsurfb.2021.112041
124. Weng T, Zhang W, Xia Y, et al., 2021, 3D bioprinting for skin tissue engineering: Current status and perspectives. J Tissue Eng, 12: 20417314211028574. https://doi.org/10.1177/20417314211028574
125. Manita PG, Garcia-Orue I, Santos-Vizcaino E, et al., 2021, 3D Bioprinting of functional skin substitutes: From current achievements to future goals. Pharmaceuticals (Basel), 14: 362. https://doi.org/10.3390/ph14040362
126. Ng WL, Wang S, Yeong WY, et al., 2016, Skin bioprinting: Impending reality or fantasy? Trends Biotechnol, 34: 689–699. https://doi.org/10.1016/j.tibtech.2016.04.006
127. Tarassoli SP, Jessop ZM, Al-Sabah A, et al., 2018, Skin tissue engineering using 3D bioprinting: An evolving research field. J Plast Reconstr Aesthet Surg, 71: 615–623. https://doi.org/10.1016/j.bjps.2017.12.006
128. Abaci HE, Guo Z, Coffman A, et al., 2016, Human skin constructs with spatially controlled vasculature using primary and iPSC-derived endothelial cells. Adv Healthc Mater, 5: 1800–1807. https://doi.org/10.1002/adhm.201500936
129. Min D, Lee W, Bae IH, et al., 2018, Bioprinting of biomimetic skin containing melanocytes. Exp Dermatol, 27: 453–459. https://doi.org/10.1111/exd.13376
130. Ng WL, Qi JT, Yeong WY, et al., 2018, Proof-of-concept: 3D bioprinting of pigmented human skin constructs. Biofabrication, 10: 025005. https://doi.org/10.1088/1758-5090/aa9e1e
131. Hong S, Song JM, 2021, A 3D cell printing-fabricated HepG2 liver spheroid model for high-content in situ quantification of drug-induced liver toxicity. Biomater Sci, 9: 5939–5950. https://doi.org/10.1039/d1bm00749a
132. Kizawa H, Nagao E, Shimamura M, et al., 2017, Scaffold-free 3D bio-printed human liver tissue stably maintains metabolic functions useful for drug discovery. Biochem Biophys Rep, 10: 186–191. https://doi.org/10.1016/j.bbrep.2017.04.004
133. Ma X, Qu X, Zhu W, et al., 2016, Deterministically patterned biomimetic human iPSC-derived hepatic model via rapid 3D bioprinting. Proc Natl Acad Sci U S A, 113: 2206–2211. https://doi.org/10.1073/pnas.1524510113
134. Faulkner-Jones A, Fyfe C, Cornelissen DJ, et al., 2015, Bioprinting of human pluripotent stem cells and their directed differentiation into hepatocyte-like cells for the generation of mini-livers in 3D. Biofabrication, 7: 044102. https://doi.org/10.1088/1758-5090/7/4/044102
135. Lei M, Wang X, 2016, Biodegradable polymers and stem cells for bioprinting. Molecules, 21: 539. https://doi.org/10.3390/molecules21050539
136. Poyck PP, Pless G, Hoekstra R, et al., 2007, In vitro comparison of two bioartificial liver support systems: MELS CellModule and AMC-BAL. Int J Artif Organs, 30: 183–191. https://doi.org/10.1177/039139880703000302
137. Calise F, Mancini A, Amoroso P, et al., 2001, Functional evaluation of the AMC-BAL to be employed in a multicentric clinical trial for acute liver failure. Transplant Proc, 33: 647–649. https://doi.org/10.1016/s0041-1345(00)02183-7
138. Nibourg GA, Hoekstra R, van der Hoeven TV, et al., 2013, Effects of acute-liver-failure-plasma exposure on hepatic functionality of HepaRG-AMC-bioartificial liver. Liver Int, 33: 516–524. https://doi.org/10.1111/liv.12090
139. van de Kerkhove MP, Poyck PP, van Wijk AC, et al., 2005, Assessment and improvement of liver specific function of the AMC-bioartificial liver. Int J Artif Organs, 28: 617–630. https://doi.org/10.1177/039139880502800611
140. van de Kerkhove MP, Poyck PP, Deurholt T, et al., 2005, Liver support therapy: an overview of the AMC-bioartificial liver research. Dig Surg, 22: 254–264. https://doi.org/10.1159/000088055
141. Kammerer S, 2021, Three-dimensional liver culture systems to maintain primary hepatic properties for toxicological analysis in vitro. Int J Mol Sci, 22: 1024. https://doi.org/10.3390/ijms221910214
142. Algahtani MS, 2021, Assessment of pharmacist’s knowledge and perception toward 3D printing technology as a dispensing method for personalized medicine and the readiness for implementation. Pharmacy (Basel), 9: 68. https://doi.org/10.3390/pharmacy9010068
143. Joseph JF, Gronbach L, Garcia-Miller J, et al., 2020, Automated real-time tumor pharmacokinetic profiling in 3D models: A novel approach for personalized medicine. Pharmaceutics, 12: 413. https://doi.org/10.3390/pharmaceutics12050413
144. Turetta M, Ben FD, Brisotto G, et al., 2018, Emerging technologies for cancer research: Towards personalized medicine with microfluidic platforms and 3D tumor models. Curr Med Chem, 25: 4616–4637. https://doi.org/10.2174/0929867325666180605122633
145. Liu Y, Chen YG, 2018, 2D-and 3D-based intestinal stem cell cultures for personalized medicine. Cells, 7: 225. https://doi.org/10.3390/cells7120225
146. Elcin YM, 2017, Special issue: Organs-on-chips & 3D-Bioprinting technologies for personalized medicine. Stem Cell Rev Rep, 13: 319–320. https://doi.org/10.1007/s12015-017-9744-2