Three-dimensional printing as a cutting-edge, versatile and personalizable vascular stent manufacturing procedure: Toward tailor-made medical devices
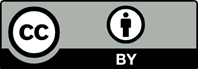
Vascular stents (VS) have revolutionized the treatment of cardiovascular diseases, as evidenced by the fact that the implantation of VS in coronary artery disease (CAD) patients has become a routine, easily approachable surgical intervention for the treatment of stenosed blood vessels. Despite the evolution of VS throughout the years, more efficient approaches are still required to address the medical and scientific challenges, especially when it comes to peripheral artery disease (PAD). In this regard, three-dimensional (3D) printing is envisaged as a promising alternative to upgrade VS by optimizing the shape, dimensions and stent backbone (crucial for optimal mechanical properties), making them customizable for each patient and each stenosed lesion. Moreover, the combination of 3D printing with other methods could also upgrade the final device. This review focuses on the most recent studies using 3D printing techniques to produce VS, both by itself and in combination with other techniques. The final aim is to provide an overview of the possibilities and limitations of 3D printing in the manufacturing of VS. Furthermore, the current situation of CAD and PAD pathologies is also addressed, thus highlighting the main weaknesses of the already existing VS and identifying research gaps, possible market niches and future directions.
1. Herrington W, Lacey B, Sherliker P, et al., 2016, Epidemiology of atherosclerosis and the potential to reduce the global burden of atherothrombotic disease. Circ Res, 118(4):535–546. https://doi.org/10.1161/CIRCRESAHA.115.307611
2. Song P, Fang Z, Wang H, et al., 2020, Global and regional prevalence, burden, and risk factors for carotid atherosclerosis: A systematic review, meta-analysis, and modelling study. Lancet Glob Health, 8(5):e721–e729. https://doi.org/10.1016/S2214-109X(20)30117-0/ ATTACHMENT/B6277D32-2818-4A94-B438- 584B317D09A4/MMC1.PDF
3. WHO, 2020, World Health Statistics 2020: Monitoring Health for the SDGs, Sustainable Development Goals. World Health Organization 2020 (WHO).
4. Poredoš P, Jug B, 2007, The prevalence of peripheral arterial disease in high risk subjects and coronary or cerebrovascular patients. Angiology, 58(3):309–315. https://doi.org/10.1177/0003319707302494
5. Bauersachs R, Zeymer U, Brière JB, et al., 2019, Burden of coronary artery disease and peripheral artery disease: A literature review. Cardiovasc Ther. 2019(8295054), 1–9. https://doi.org/10.1155/2019/8295054
6. Virani SS, Alonso A, Aparicio HJ, et al., 2021, Heart disease and stroke statistics—2021 update. Circulation, 143:E254– E743. https://doi.org/10.1161/CIR.0000000000000950
7. Juchniewicz H, Lubkowska A, 2020, Oxygen-ozone (O2- O3) therapy in peripheral arterial disease (PAD): A review study. Ther Clin Risk Manag, 16:579. https://doi.org/10.2147/TCRM.S255247
8. Ouriel K, Caves KM, Chaikof EL, et al., 2006, The evolving impact of microfabrication and nanotechnology on stent design. J Vasc Surg, 44:1363–1368. https://doi.org/10.1016/j.jvs.2006.08.046
9. IMARC, 2022, Vascular stents market: Global industry trends, share, size, growth, oportunity and forecast 2022- 2027. Accessed: April 09, 2022. [Online]. Available. https://www.imarcgroup.com/vascular-stents-market
10. Grüntzig A, 1978, Transluminal dilatation of coronary-artery stenosis. Lancet (London, England), 1(8058):263. https://doi.org/10.1016/S0140-6736(78)90500-7
11. Lobato EB, 2019, Care of the patient with coronary stents undergoing noncardiac surgery, in Essentials of Cardiac Anesthesia for Noncardiac Surgery: A Companion to Kaplan’s Cardiac Anesthesia, J. A. Kaplan, Ed. China, Elsevier, 33–69.
12. Gao G, Yi H-G, Jiang W, et al., 2022, A review on manufacturing and post-processing technology of vascular stents. Micromachines, 13(1):1–16. https://doi.org/10.3390/MI13010140
13. Uhlemann M, Möbius-Winkler S, Adam J, et al., 2016, The Leipzig prospective drug-eluting balloon-registry – outcome of 484 consecutive patients treated for coronary in-stent restenosis and de novo lesions using paclitaxel-coated balloons. Circ J, 80(2): CJ-14-1352. https://doi.org/10.1253/CIRCJ.CJ-14-1352
14. Lee SJ, Jo HH, Lim KS, et al., 2019, Heparin coating on 3D printed poly (L-lactic acid) biodegradable cardiovascular stent via mild surface modification approach for coronary artery implantation. Chem Eng J, 378(122116):1–15. https://doi.org/10.1016/J.CEJ.2019.122116
15. Beshchasna N, Saqib M, Kraskiewicz H, et al., 2020, Recent advances in manufacturing innovative stents. Pharmaceutics, 12(349):1–36.
16. Fontaine AB, Koelling K, Clay J, et al., 1994, Decreased platelet adherence of polymer-coated tantalum stents. J Vasc Interv Radiol, 5(4):567–572. https://doi.org/10.1016/S1051-0443(94)71555-4
17. Borhani S, Hassanajili S, Tafti SHA, et al., 2018, Cardiovascular stents: Overview, evolution, and next generation. Prog Biomater 2018 73, 7(3):175–205. https://doi.org/10.1007/S40204-018-0097-Y
18. Ooi OC, Mullany CJ, Rihal CS, 2007, Revascularization options for ischemic heart disease: Coronary artery bypass grafting and percutaneous coronary intervention, in Cardiovascular Therapeutics: A Companion to Braunwald’s Heart Disease, 3rd ed., E. M. Antman and M. S. Sabatine, Eds. W.B. Saunders, 157–177.
19. Scoutaris N, Chai F, Maurel B, et al., 2015, Development and biological evaluation of inkjet printed drug coatings on intravascular stent. Mol Pharm, 13(1):125–133. https://doi.org/10.1021/ACS.MOLPHARMACEUT.5B00570
20. Krankenberg H, et al., 2017, Self-expanding versus balloon-expandable stents for iliac artery occlusive disease: The randomized ICE trial. JACC Cardiovasc Interv, 10(16):1694– 1704. https://doi.org/10.1016/J.JCIN.2017.05.015
21. Fornell D, 2019, Trends in coronary, carotid and peripheral stents | DAIC. Diagn Interv Cardiol. https://www.dicardiology.com/article/trends-coronary-carotid-and-peripheral-stents (Accessed April 02, 2022)
22. van Haelst STW, Peeters Weem SMO, Moll FL, et al., 2016, Current status and future perspectives of bioresorbable stents in peripheral arterial disease. J Vasc Surg, 64(4): 1151–1159.e1. https://doi.org/10.1016/J.JVS.2016.05.044
23. Medical Advisory Secretariat, 2010, Stenting for peripheral artery disease of the lower extremities: An evidence-based analysis. Ont Health Technol. Assess Ser, 10(18):1–88. Accessed: April 02, 2022 [Online]. Available. https://pubmed.ncbi.nlm.nih.gov/23074395/
24. Klabunde RE, 2017, Coronary anatomy and blood flow. Cardiovasc Physiol Concepts. https://www.cvphysiology.com/Blood Flow/BF001 (Accessed April 02, 2022).
25. Klabunde RE, 2010, Autoregulation of organ blood flow. Cardiovas Physiol Concepts. https://www.cvphysiology.com/Blood Flow/BF004 (Accessed April 02, 2022).
26. Mani G, Feldman MD, Patel D, et al., 2007, Coronary stents: A materials perspective. Biomaterials, 28(9):1689–1710. https://doi.org/10.1016/J.BIOMATERIALS.2006.11.042
27. Lachowitzer M, 2008, Assessing radial tests for endovascular implants. Med Device Diagn Ind. https://www.mddionline.com/news/assessing-radial-tests-endovascular-implants (Accessed February 02, 2022).
28. Stoeckel D, Bonsignore C, Duda S, 2002, A survey of stent designs. Minim Invasive Ther Allied Technol, 11(4):137–147. Accessed: February 02, 2022 [Online]. Available. https://sci-hub.ru/https://www.tandfonline.com/doi/ abs/10.1080/136457002760273340
29. Abhyankar AD, Thakkar AS, 2012, In vivo assessment of stent recoil of biodegradable polymer-coated cobalt– chromium sirolimus-eluting coronary stent system. Indian Heart J, 64(6):546. https://doi.org/10.1016/J.IHJ.2012.07.005
30. Lee MS, Banka G, 2016, In-stent restenosis. Interv Cardiol Clin, 5(2):211–220. https://doi.org/10.1016/J.ICCL.2015.12.006
31. Modi K, Soos MP, Mahajan K, 2021, Stent thrombosis. StatPearls 1–15. Accessed: February 03, 2022 [Online]. Available. https://www.ncbi.nlm.nih.gov/books/NBK441908/
32. Reejhsinghani R, Lotfi AS, 2015, Prevention of stent thrombosis: Challenges and solutions. Vasc Health Risk Manag, 2015(11):93–106. https://doi.org/10.2147/VHRM.S43357
33. Kolandaivelu K, Swaminathan R, Gibson WJ, et al., 2011, Stent thrombogenicity early in high-risk interventional settings is driven by stent design and deployment and protected by polymer-drug coatings. Circulation, 123(13):1400–1409. https://doi.org/10.1161/CIRCULATIONAHA.110.003210
34. Hotta E, Hara H, Kamiya T, et al., 2018, Non-thermal atmospheric pressure plasma-induced IL-8 expression is regulated via intracellular K + loss and subsequent ERK activation in human keratinocyte HaCaT cells. Arch Biochem Biophys, 644:64–71. https://doi.org/10.1016/j.abb.2018.03.005
35. Cvrček L, Horáková M, 2019, Plasma modified polymeric materials for implant applications, in Non-Thermal Plasma Technology for Polymeric Materials. Applications in Composites, Nanostructured Materials and Biomedical Fields., S. Thomas, M. Mozetič, U. Cvelbar, et al., Eds. Elsevier, 367–407.
36. Guo R, He Y, Li H, et al., 2020, Development of 3D-printed sulfated chitosan modified bioresorbable stents for coronary artery disease. Front Bioeng Biotechnol, 8(462):1–12. https://doi.org/10.3389/fbioe.2020.00462
37. Demir AG, Previtali B, 2017, Additive manufacturing of cardiovascular CoCr stents by selective laser melting. Mater Des, 119:338–350. https://doi.org/10.1016/J.MATDES.2017.01.091
38. Zou Q, Xue W, Lin J, et al., 2016, Mechanical characteristics of novel polyester/NiTi wires braided composite stent for the medical application. Results Phys, 6:440–446. https://doi.org/10.1016/J.RINP.2016.07.007
39. Ahlhelm F, Kaufmann R, Ahlhelm D, et al., 2009, Carotid artery stenting using a novel self-expanding braided nickel-titanium stent: Feasibility and safety porcine trial. Cardiovasc Interv Radiol, 32(5):1019–1027. https://doi.org/10.1007/S00270-009-9572-0
40. Ueng KC, Wen SP, Lou CW, et al., 2016, Stainless steel/nitinol braid coronary stents: Braiding structure stability and cut section treatment evaluation. J Ind Text, 45(5):965–977. https://doi.org/10.1177/1528083714550054
41. Marti P, Lampus F, Benevento D, et al., 2019, Trends in use of 3D printing in vascular surgery: A survey. Int Angiol, 38(5):418–424. https://doi.org/10.23736/S0392-9590.19.04148-8
42. Memon S, Friend E, Samuel SP, et al., 2021, 3D printing of carotid artery and aortic arch anatomy: Implications for preprocedural planning and carotid stenting. J Invasive Cardiol, 33(9):E723–E729. Accessed: December 30, 2021 [Online]. Available. https://pubmed.ncbi.nlm.nih.gov/34473073/
43. Valverde I, Gomez G, Coserria JF, et al., 2015, 3D printed models for planning endovascular stenting in transverse aortic arch hypoplasia. Catheter Cardiovasc Interv, 85(6):1006–1012. https://doi.org/10.1002/CCD.25810
44. Sun Z, Jansen S, 2019, Personalized 3D printed coronary models in coronary stenting. Quant Imaging Med Surg, 9(8):1356–1367. https://doi.org/10.21037/QIMS.2019.06.21
45. Bortman J, Mahmood F, Schermerhorn M, et al., 2019, Use of 3-dimensional printing to create patient-specific abdominal aortic aneurysm models for preoperative planning. J Cardiothorac Vasc Anesth, 33(5):1442–1446. https://doi.org/10.1053/J.JVCA.2018.08.011
46. Barón V, Guevara R, 2019, Three-dimensional printing-guided fenestrated endovascular aortic aneurysm repair using open source software and physician-modified devices. J Vasc Surg Cases Innov Tech, 5(4):566–571. https://doi.org/10.1016/J.JVSCIT.2019.08.006
47. Young L, Harb SC, Puri R, et al., 2020, Percutaneous coronary intervention of an anomalous coronary chronic total occlusion: The added value of three-dimensional printing. Catheter Cardiovasc Interv, 96(2):330–335. https://doi.org/10.1002/CCD.28625
48. Walker JL, Santoro M, 2017, Processing and production of bioresorbable polymer scaffolds for tissue engineering, in Bioresorbable Polymers for Biomedical Applications: From Fundamentals to Translational Medicine, G. Perale and J. Hilborn, Eds. Woodhead Publishing, 181–203.
49. Singh R, Singh S, Hashmi MSJ, 2016, Implant materials and their processing technologies, in Materials Science and Materials Engineering, Elsevier.
50. Mohapatra S, Kar RK, Biswal PK, et al., 2022, Approaches of 3D printing in current drug delivery. Sensors Int, 3(100146): 1–10. https://doi.org/10.1016/J.SINTL.2021.100146
51. Schwab A, Levato R, D’Este M, et al., 2020, Printability and shape fidelity of bioinks in 3D bioprinting. Chem Rev, 120(19):11028–11055. https://doi.org/10.1021/acs.chemrev.0c00084
52. Garcia-Villen F, Ruiz-Alonso S, Lafuente-Merchan M, et al., 2021, Clay minerals as bioink ingredients for 3D printing and 3D bioprinting: Application in tissue engineering and regenerative medicine. Pharmaceutics, 13(1806):1–46. [Online]. Available. https://pubmed.ncbi.nlm.nih.gov/34834221/
53. Manita PG, Garcia-Orue I, Santos-Vizcaino E, et al., 2021, 3D bioprinting of functional skin substitutes: From current achievements to future goals. Pharmaceuticals, 14(362): 1–25. [Online]. Available. https://www.mdpi.com/1424-8247/14/4/362
54. Jamee R, Araf Y, Bin Naser I, et al., 2021, The promising rise of bioprinting in revolutionalizing medical science: Advances and possibilities. Regen Ther, 18:133–145. https://doi.org/10.1016/J.RETH.2021.05.006
55. Begum S, Karim ANM, Ansari MNM, et al., 2020, Nanomaterials, in Encyclopedia of Renewable and Sustainable Materials, vol. 1, S. Hashmi and I. A. Choudhury, Eds. Elsevier, 515–539.
56. Song X, Zhai W, Huang R, et al., 2022, Metal-based 3D-printed micro parts & structures, in Encyclopedia of Materials: Metals and Alloys, vol. 4, F. G. Caballero, Ed. Elsevier, 448–461.
57. Zhu C, Liu T, Qian F, et al., 2017, 3D printed functional nanomaterials for electrochemical energy storage. Nano Today, 15:107–120. https://doi.org/10.1016/J.NANTOD.2017.06.007
58. Xu C, Bouchemit A, L’Espérance G, et al., 2017, Solvent-cast based metal 3D printing and secondary metallic infiltration. J Mater Chem C, 5(40):10448–10455. https://doi.org/10.1039/C7TC02884A
59. Guerra AJ, Cano P, Rabionet M, et al., 2018, 3D-printed PCL/PLA composite stents: Towards a new solution to cardiovascular problems. Materials (Basel, Switzerland), 11(9): 1–13. https://doi.org/10.3390/MA11091679
60. Guerra AJ, Ciurana J, 2018, 3D-printed bioabsordable polycaprolactone stent: The effect of process parameters on its physical features. Mater Design, 137:430–437. https://doi.org/10.1016/J.MATDES.2017.10.045
61. Wu Z, Zhao J, Wu W, et al., 2017, A novel 3D additive manufacturing machine to biodegradable stents. Proc Manuf, 13:718–723. https://doi.org/10.1016/J.PROMFG.2017.09.118
62. Wu Z, et al., 2018, Radial compressive property and the proof-of-concept study for realizing self-expansion of 3D printing polylactic acid vascular stents with negative Poisson’s ratio structure. Materials (Basel), 11(8):1357. https://doi.org/10.3390/MA11081357
63. Jia H, Gu SY, Chang K, 2018, 3D printed self-expandable vascular stents from biodegradable shape memory polymer. Adv Polym Technol, 37(8):3222–3228. https://doi.org/10.1002/ADV.22091
64. Zhang C, Cai D, Liao P, et al., 2021, 4D printing of shape-memory polymeric scaffolds for adaptive biomedical implantation. Acta Biomater, 122:101–110. https://doi.org/10.1016/J.ACTBIO.2020.12.042
65. Kim D, Kim T, Lee YG, 2019, 4D printed bifurcated stents with kirigami-inspired structures. J Vis Exp, 149(e59746):1–9. https://doi.org/10.3791/59746
66. Kim T, Lee Y-G, 2018, Shape transformable bifurcated stents. Sci Rep, 8(13911):1–9. https://doi.org/10.1038/s41598-018-32129-3
67. Zhang Y, Zhao J, Yang G, et al., 2019, Mechanical properties and degradation of drug eluted bioresorbable vascular scaffolds prepared by three-dimensional printing technology. J Biomater Sci Polym Ed, 30(7):547–560. https://doi.org/10.1080/09205063.2019.1586303
68. Lei Y, Chen X, Li Z, et al., 2020, A new process for customized patient-specific aortic stent graft using 3D printing technique. Med Eng Phys, 77:80–87. https://doi.org/10.1016/j.medengphy.2019.12.002
69. Park SA, Lee SJ, Lim KS, et al., 2015, In vivo evaluation and characterization of a bio-absorbable drug-coated stent fabricated using a 3D-printing system. Mater Lett, 141: 355–358. https://doi.org/10.1016/J.MATLET.2014.11.119
70. Misra SK, Ostadhossein F, Babu R, et al., 2017, 3D-printed multidrug-eluting stent from graphene-nanoplatelet-doped biodegradable polymer composite. Adv Healthc Mater, 6(1700008):1–14. https://doi.org/10.1002/ADHM.201700008
71. Zhou Y, Zhou D, Cao P, et al., 2021, 4D printing of shape memory vascular stent based on βCD-g-polycaprolactone. Macromol Rapid Commun, 42(14):2100176. https://doi.org/10.1002/MARC.202100176
72. Singh J, Kaur T, Singh N, et al., 2020, Biological and mechanical characterization of biodegradable carbonyl iron powder/polycaprolactone composite material fabricated using three-dimensional printing for cardiovascular stent application. Proc Inst Mech Eng H, 234(9):975–987. https://doi.org/10.1177/0954411920936055
73. Van Lith R, Baker E, Ware H, et al., 2016, 3D-printing strong high-resolution antioxidant bioresorbable vascular stents. Adv Mater Technol, 1(9): 1–7. https://doi.org/10.1002/ADMT.201600138
74. Ware HOT, Farsheed AC, Akar B, et al., 2018, High-speed on-demand 3D printed bioresorbable vascular scaffolds. Mater Today Chem, 7:25–34. https://doi.org/10.1016/J.MTCHEM.2017.10.002
75. de Oliveira MF, da Silva LCE, de Oliveira MG, 2021, 3D printed bioresorbable nitric oxide-releasing vascular stents. Bioprinting, 22(e00137): 1–10. https://doi.org/10.1016/J.BPRINT.2021.E00137
76. Yang J, Webb AR, Pickerill SJ, et al., 2006, Synthesis and evaluation of poly(diol citrate) biodegradable elastomers. Biomaterials, 27(9):1889–1898. https://doi.org/10.1016/J.BIOMATERIALS.2005.05.106
77. Ismaeel A, Papoutsi E, Miserlis D, et al., 2020, The nitric oxide system in peripheral artery disease: Connection with oxidative stress and biopterins. Antioxidants, 9(7):1–16. https://doi.org/10.3390/ANTIOX9070590
78. Flege C, Vogt F, Höges S, et al., 2013, Development and characterization of a coronary polylactic acid stent prototype generated by selective laser melting. J Mater Sci Mater Med, 24(1):241–255. https://doi.org/10.1007/S10856-012-4779-Z
79. Elliott MR, Kim D, Molony DS, et al., 2019, Establishment of an automated algorithm utilizing optical coherence tomography and micro-computed tomography imaging to reconstruct the 3-D deformed stent geometry. IEEE Trans Med Imaging, 38(3):710–720. https://doi.org/10.1109/TMI.2018.2870714
80. Wiesent L, Spear A, Nonn A, 2022, Computational analysis of the effects of geometric irregularities on the interaction of an additively manufactured 316L stainless steel stent and a coronary artery. J Mech Behav Biomed Mater, 125:1–12. https://doi.org/10.1016/J.JMBBM.2021.104878
81. Chiastra C, Mazzi V, Lodi Rizzini M, et al., 2022, Coronary artery stenting affects wall shear stress topological skeleton. J Biomech Eng. 144(6):061002, 1–11. https://doi.org/10.1115/1.4053503
82. Pan C, Han Y, Lu J, 2021, Structural design of vascular stents: A review. Micromachines, 12(7):770.
https://doi.org/10.3390/MI12070770
83. Habib A, Finn AV, 2015, Endothelialization of drug eluting stents and its impact on dual anti-platelet therapy duration. Pharmacol Res, 93:22–27. https://doi.org/10.1016/J.PHRS.2014.12.003
84. Nguyen DT, Smith AF, Jiménez JM, 2021, Stent strut streamlining and thickness reduction promote endothelialization. J R Soc Interface, 18(181):1–14. https://doi.org/10.1098/RSIF.2021.0023
85. Cabrera MS, Sanders B, Goor OLGM, et al., 2017, Computationally designed 3D printed self-expandable polymer stents with biodegradation capacity for minimally invasive heart valve implantation: a proof-of-concept study. 3D Print Addit Manuf, 4(1):19–29. https://doi.org/10.1089/3DP.2016.0052/ASSET/IMAGES/ LARGE/FIGURE8.JPEG
86. Vellayappan MV, Balaji A, Subramanian AP, et al., 2015, Multifaceted prospects of nanocomposites for cardiovascular grafts and stents. Int J Nanomed, 10:2785–2803. https://doi.org/10.2147/IJN.S80121
87. Chou TC, Fu E, Wu CJ, et al., 2003, Chitosan enhances platelet adhesion and aggregation. Biochem Biophys Res Commun, 302(3):480–483. https://doi.org/10.1016/S0006-291X(03)00173-6
88. Wickham AM, Islam MM, Mondal D, et al., 2014, Polycaprolactone–thiophene-conjugated carbon nanotube meshes as scaffolds for cardiac progenitor cells. J Biomed Mater Res Part B Appl Biomater, 102(7):1553–1561. https://doi.org/10.1002/JBM.B.33136
89. Stout DA, Yoo J, Santiago-Miranda AN, et al., 2012, Mechanisms of greater cardiomyocyte functions on conductive nanoengineered composites for cardiovascular application. Int J Nanomed, 7:5653–5669. https://doi.org/10.2147/IJN.S34574
90. Mattioli-Belmonte M, Vozzi G, Whulanza Y, et al., 2012, Tuning polycaprolactone–carbon nanotube composites for bone tissue engineering scaffolds. Mater Sci Eng C, 32(2):152–159. https://doi.org/10.1016/J.MSEC.2011.10.010
91. Chakoli AN, Wan J, Feng JT, et al., 2009, Functionalization of multiwalled carbon nanotubes for reinforcing of poly(l-lactide-co-ε-caprolactone) biodegradable copolymers. Appl Surf Sci, 256(1):170–177. https://doi.org/10.1016/J.APSUSC.2009.07.103
92. Lee HH, Shin US, Jin GZ, et al., 2011, Highly homogeneous carbon nanotube-polycaprolactone composites with various and controllable concentrations of ionically-modified- MWCNTs. Bull Korean Chem Soc, 32(1):157–161. https://doi.org/10.5012/BKCS.2011.32.1.157
93. Arabi H, Mirzadeh H, Ahmadi SH, et al., 2004, In vitro and in vivo hemocompatibility evaluation of graphite coated polyester vascular grafts. Int J Artif Organs 27(8):691–698. https://doi.org/10.1177/039139880402700807
94. Podila R, Moore T, Alexis F, et al., 2013, Graphene coatings for biomedical implants. J Vis Exp, 73(e50276):1–9. https://doi.org/10.3791/50276
95. Gao F, Hu Y, Li G, et al., 2020, Layer-by-layer deposition of bioactive layers on magnesium alloy stent materials to improve corrosion resistance and biocompatibility. Bioact Mater, 5(3):611–623. https://doi.org/10.1016/J.BIOACTMAT.2020.04.016
96. Yang MC, Tsou HM, Hsiao YS, et al., 2019, Electrochemical polymerization of PEDOT-graphene oxide-heparin composite coating for anti-fouling and anti-clotting of cardiovascular stents. Polymers (Basel), 11(9):1–15. https://doi.org/10.3390/POLYM11091520
97. Alshebly YS, Nafea M, Mohamed Ali MS, et al., 2021, Review on recent advances in 4D printing of shape memory polymers. Eur Polym J, 159:110708. https://doi.org/10.1016/J.EURPOLYMJ.2021.110708
98. Melocchi A, Uboldi M, Cerea M, et al., 2021, Shape memory materials and 4D printing in pharmaceutics. Adv Drug Deliv Rev, 173:216–237. https://doi.org/10.1016/J.ADDR.2021.03.013
99. Xu J, Zhang Y, Feng YB, et al., 2018, Electromagnetic and mechanical properties of carbonyl iron powder-filled methyl vinyl silicone rubber during thermal aging. Polym Compos, 39(8):2897–2903. https://doi.org/10.1002/PC.24286
100. Waksman R, Pakala R, Baffour R, et al., 2008, Short-term effects of biocorrodible iron stents in porcine coronary arteries. J Interv Cardiol, 21(1):15–20. https://doi.org/10.1111/J.1540-8183.2007.00319.X
101. Peuster M, Hesse C, Schloo T, et al., 2006, Long-term biocompatibility of a corrodible peripheral iron stent in the porcine descending aorta. Biomaterials, 27(28):4955–4962. https://doi.org/10.1016/J.BIOMATERIALS.2006.05.029
102. Peuster M, Wohlsein P, Brügmann M, et al., 2001, A novel approach to temporary stenting: Degradable cardiovascular stents produced from corrodible metal-results 6-18 months after implantation into New Zealand white rabbits. Heart, 86(5):563–569. https://doi.org/10.1136/HEART.86.5.563
103. Liu B, Zheng YF, 2011, Effects of alloying elements (Mn, Co, Al, W, Sn, B, C and S) on biodegradability and in vitro biocompatibility of pure iron. Acta Biomater, 7(3):1407–1420. https://doi.org/10.1016/J.ACTBIO.2010.11.001
104. Khoo ZX, Liu Y, An J, et al., 2018, A review of selective laser melted NiTi shape memory alloy. Material, 11:519. https://doi.org/10.3390/MA11040519
105. Biffi CA, Fiocchi J, Valenza F, 2020, et al., Selective laser melting of NiTi shape memory alloy: Processability, microstructure, and superelasticity. Shape Mem Superelasticity, 6(3):342–353. https://doi.org/10.1007/S40830-020-00298-8/FIGURES/11
106. Safavi MS, Bordbar‐Khiabani A, Khalil‐allafi J, et al., 2022, Additive manufacturing: An opportunity for the fabrication of near‐net‐shape NiTi implants. J Manuf Mater Process, 6(3):1–22. https://doi.org/10.3390/JMMP6030065
107. Hassani FA, Peh WYX, Gammad GGL, et al., 2017, A 3D printed implantable device for voiding the bladder using shape memory alloy (SMA) actuators. Adv Sci, 4(11):1–10. https://doi.org/10.1002/ADVS.201700143
108. Tong A, Pham QL, Abatemarco P, et al., 2021, Review of low-cost 3D bioprinters: State of the market and observed future trends. SLAS Technol, 26(4):333–366. https://doi.org/10.1177/24726303211020297
109. Choonara YE, Du Toit LC, Kumar P, et al., 2016, 3D-printing and the effect on medical costs: A new era? Expert Rev Pharmacoecon Outcomes Res, 16(1):23–32. https://doi.org/10.1586/14737167.2016.1138860
110. van Tonder L, Labuschagné FJWJ, 2021, Systematic literature review of the effect of layered double hydroxides on the mechanical properties of rubber. Polymer, 13:3716. https://doi.org/10.3390/POLYM13213716
111. Coppola B, Cappetti N, Di Maio L, et al., 2017, Layered silicate reinforced polylactic acid filaments for 3D printing of polymer nanocomposites, 1–4. https://doi.org/10.1109/RTSI.2017.8065892
112. Papageorgiou DG, Li Z, Liu M, et al., 2020, Mechanisms of mechanical reinforcement by graphene and carbon nanotubes in polymer nanocomposites. Nanoscale, 12(4. Royal Society of Chemistry): 2228–2267. https://doi.org/10.1039/c9nr06952f
113. Coleman JN, Khan U, Gun’ko YK, 2006, Mechanical reinforcement of polymers using carbon nanotubes. Adv Mater, 18(6):689–706. https://doi.org/10.1002/ADMA.200501851
114. Kim H, Ryu KH, Baek D, et al., 2020, 3D printing of polyethylene terephthalate glycol-sepiolite composites with nanoscale orientation. ACS Appl Mater Interfaces, 12(20):23453–23463. https://doi.org/10.1021/acsami.0c03830
115. Zhou K, Dey M, Ayan B, et al., 2021, Fabrication of PDMS microfluidic devices using nanoclay-reinforced Pluronic F-127 as a sacrificial ink. Biomed Mater, 16(4):45005. https://doi.org/10.1088/1748-605X/abe55e
116. Gu Z, Rolfe BE, Xu ZP, et al., 2012, Antibody-targeted drug delivery to injured arteries using layered double hydroxide nanoparticles. Adv Healthc Mater, 1(5):669–673. https://doi.org/10.1002/ADHM.201200069
117. Gu Z, Rolfe B, Thomas A, et al., 2013, Restenosis treatments using nanoparticle-based drug delivery systems. Curr Pharm Des, 19(35):6330–6339. https://doi.org/10.2174/1381612811319350009
118. Gu Z, Rolfe BE, Xu ZP, et al., 2010, Enhanced effects of low molecular weight heparin intercalated with layered double hydroxide nanoparticles on rat vascular smooth muscle cells. Biomaterials, 31(20):5455–5462. https://doi.org/10.1016/J.BIOMATERIALS.2010.03.050
119. Ishihara S, Machino T, Deguchi K, et al., 2021, Disposable nitric oxide generator based on a structurally deformed nitrite-type layered double hydroxide. Inorg Chem, 60(21):16008–16015. https://doi.org/10.1021/ACS.INORGCHEM.1C00456
120. Russell SE, González Carballo JM, Orellana-Tavra C, et al., 2017, A comparison of copper and acid site zeolites for the production of nitric oxide for biomedical applications. Dalt Trans, 46(12):3915–3920. https://doi.org/10.1039/C7DT00195A
121. Doyle RA, Russell SE, Morris RE, 2019, Nitric oxide production from nitrite by a series of zeolites produced via the ADOR route. Microporous Mesoporous Mater, 280:367–371. https://doi.org/10.1016/J.MICROMESO.2019.02.019
122. Jung SY, Kim HM, Hwang S, et al., 2020, Physicochemical properties and hematocompatibility of layered double hydroxide-based anticancer drug methotrexate delivery system. Pharm, 12:1210. https://doi.org/10.3390/PHARMACEUTICS12121210
123. Gu Z, Yan S, Cheong S, et al., 2018, Layered double hydroxide nanoparticles: Impact on vascular cells, blood cells and the complement system. J Colloid Interface Sci, 512:404–410. https://doi.org/10.1016/J.JCIS.2017.10.069
124. Vaiana CA, Leonard MK, Drummy LF, et al., 2011, Epidermal growth factor: Layered silicate nanocomposites for tissue regeneration. Biomacromolecules, 12(9):3139–3146. https://doi.org/10.1021/bm200616v
125. Wu K, Feng R, Jiao Y, et al., 2017, Effect of halloysite nanotubes on the structure and function of important multiple blood components. Mater Sci Eng C, 75:72–78. https://doi.org/10.1016/J.MSEC.2017.02.022
126. Devlin JJ, Kircher S, Kozen BG, et al., 2011, Comparison of ChitoFlex®, CELOXTM, and QuikClot® in control of hemorrhage. J Emerg Med, 41(3):237–245. ht t p s : / / d o i . o r g / ht t p : / / d x . d o i . o r g / 1 0 . 1 0 1 6 / j . jemermed.2009.02.017
127. Ran Y, et al., 2010, QuikClot combat gauze use for hemorrhage control in military trauma: January 2009 Israel Defense Force Experience in the Gaza Strip—A preliminary report of 14 cases. Prehosp Disaster Med, 25(6):584–588 [Online]. Available: ht t p : / / journa l s . cambr i d ge. o rg / a c t i on / d isplay Abstract?fromPage=online&aid=8257157&fileId= S1049023X00008797
128. Choi SJ, Oh JM, Choy JH, 2008, Safety aspect of inorganic layered nanoparticles: Size-dependency in vitro and in vivo. J Nanosci Nanotechnol, 8(10):5297–5301. https://doi.org/10.1166/JNN.2008.1143
129. Tahmasebi-Birgani Z, Solati-Hashjin M, Peirovi H, et al., 2010, Layered double hydroxide: A new ceramid-based hemostatic agent?, in Ceramic Transactions, vol. 218, R. Narayan, M. Singh, and McKittrick, Eds. Wiley-American Ceramic Society, 53–57.
130. Mahesh V, Joseph AS, Mahesh V, et al., 2021, Investigation on the mechanical properties of additively manufactured PETG composites reinforced with OMMT nanoclay and carbon fibers. Polym Compos, 42:2380–2395. https://doi.org/10.1002/pc.25985
131. Coppola B, Cappetti N, Di Maio L, et al., 2018, 3D printing of PLA/clay nanocomposites: Influence of printing temperature on printed samples properties. Materials (Basel), 11(1947):1–17. https://doi.org/10.3390/ma11101947
132. Paspali A, Bao Y, Gawne DT, et al., 2018, The influence of nanostructure on the mechanical properties of 3D printed polylactide/nanoclay composites. Compos Part B Eng, 152:160–168. https://doi.org/10.1016/j.compositesb.2018.07.005
133. Adepu S, Luo H, Ramakrishna S, 2021, Heparin-tagged PLA-PEG copolymer-encapsulated biochanin A-loaded (Mg/Al) LDH nanoparticles recommended for non-thrombogenic and anti-proliferative stent coating. Int J Mol Sci, 22(11):5433. https://doi.org/10.3390/IJMS22115433/S1
134. Xiao R, Huang WM, Xiao R, et al., 2020, Heating/solvent responsive shape-memory polymers for implant biomedical devices in minimally invasive surgery: Current status and challenge. Macromol Biosci, 20(8):2000108. https://doi.org/10.1002/MABI.202000108
135. Bhatia M, Bhatia S, Siddhartha, 2022, Smart materials for cardiovascular devices. Mater Today Proc, 53:307–309. https://doi.org/10.1016/J.MATPR.2021.12.591
136. Jiang JJ, Hu Y, Chen X, et al., 2018, Development and application of shape memory intelligent composites. Cailiao Gongcheng/J Mater Eng, 46(8):1–13. https://doi.org/10.11868/J.ISSN.1001-4381.2018.000344
137. Hua W, Shi W, Mitchell K, et al., 2022, 3D printing of biodegradable polymer vascular stents: A review. Chin J Mech Eng Addit Manuf Front, 1(2):100020. https://doi.org/10.1016/J.CJMEAM.2022.100020
138. Kluska E, Gruda P, Majca-Nowak N, 2018, The accuracy and the printing resolution comparison of different 3D printing technologies. Trans Aerosp Res, 2018(3):69–86. https://doi.org/10.2478/TAR-2018-0023
139. Nulty A, 2022, A comparison of trueness and precision of 12 3D printers used in dentistry. BDJ Open, 8(1):1–9. https://doi.org/10.1038/s41405-022-00108-6
140. Chae MP, Chung RD, Smith JA, et al., 2021, The accuracy of clinical 3D printing in reconstructive surgery: Literature review and in vivo validation study. Gland Surg, 10(7): 2293. https://doi.org/10.21037/GS-21-264
141. Ho Kim J, Pinhata-Baptista OH, Ayres AP, et al., 2022, Accuracy comparison among 3D-printing technologies to produce dental models. Appl Sci, 12(8425):1–9.
142. Nguyen PD, Nguyen TQ, Tao QB, et al., 2022, A data-driven machine learning approach for the 3D printing process optimisation. Virtual Phys Prototyp 17(4):768–786. https://doi.org/10.1080/17452759.2022.2068446
143. Gong X, Zeng D, Groeneveld-Meijer W, et al., 2022, Additive manufacturing: A machine learning model of process-structure-property linkages for machining behavior of Ti- 6Al-4V. Mater Sci Addit Manuf, 1(1):6. https://doi.org/10.18063/MSAM.V1I1.6
144. Sing SL, Kuo CN, Shih CT, et al., 2021, Perspectives of using machine learning in laser powder bed fusion for metal additive manufacturing. Virtual Phys Prototyp 16(3):372–386. https://doi.org/10.1080/17452759.2021.1944229
145. Smolderen KG, Wang K, De Pouvourville G, et al., 2012, Two-year vascular hospitalisation rates and associated costs in patients at risk of atherothrombosis in France and Germany: Highest burden for peripheral arterial disease. Eur J Vasc Endovasc Surg, 43(2):198–207. https://doi.org/10.1016/J.EJVS.2011.09.016