Experimental study on repair of cartilage defects in the rabbits with GelMA-MSCs scaffold prepared by three-dimensional bioprinting
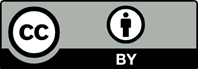
Cartilage damage is a common orthopedic disease, which can be caused by sports injury, obesity, joint wear, and aging, and cannot be repaired by itself. Surgical autologous osteochondral grafting is often required in deep osteochondral lesions to avoid the later progression of osteoarthritis. In this study, we fabricated a gelatin methacryloylmarrow mesenchymal stem cells (GelMA-MSCs) scaffold by three-dimensional (3D) bioprinting. This bioink is capable of fast gel photocuring and spontaneous covalent cross-linking, which can maintain high viability of MSCs and provide a benign microenvironment to promote the interaction, migration, and proliferation of cells. In vivo experiments, further, proved that the 3D bioprinting scaffold can promote the regeneration of cartilage collagen fibers and have a remarkable effect on cartilage repair of rabbit cartilage injury model, which may represent a general and versatile strategy for precise engineering of cartilage regeneration system.
1. Chen D, Shen J, Zhao W, et al., 2017, Osteoarthritis: toward a comprehensive understanding of pathological mechanism. Bone Res, 5: 16044. https://doi.org/10.1038/boneres.2016.44
2. Decker RS. Articular cartilage and joint development from embryogenesis to adulthood. Semin Cell Dev Biol, 62: 50–56. https://doi.org/10.1016/j.semcdb.2016.10.005
3. Chen S, Fu P, Wu H, et al., 2017, Meniscus, articular cartilage and nucleus pulposus: A comparative review of cartilage-like tissues in anatomy, development and function. Cell Tissue Res, 370: 53–70. https://doi.org/10.1007/s00441-017-2613-0
4. Campos Y, Almirall A, Fuentes G, et al., 2019, Tissue engineering: An alternative to repair cartilage. Tissue Eng Part B Rev, 25: 357–373. https://doi.org/10.1089/ten.TEB.2018.0330
5. Lories RJ, Luyten FP, 2011, The bone-cartilage unit in osteoarthritis. Nat Rev Rheumatol, 7: 43–49. https://doi.org/10.1038/nrrheum.2010.197
6. Murphy SV, De Coppi P, Atala A. Opportunities and challenges of translational 3D bioprinting. Nat Biomed Eng, 4: 370–380. https://doi.org/10.1038/s41551-019-0471-7
7. Vanaei A, Parizi M, 2021, An overview on materials and techniques in 3D bioprinting toward biomedical application. Engin Regen, 2: 1–18. https://doi.org/10.1088/1748-6041/11/2/022001
8. Zhang D, Chen Q, Shi C, 2020, Dealing with the foreign-body response to implanted biomaterials: Strategies and applications of new materials. Adv Funct Mater, 31: 2007226. https://doi.org/10.1002/adfm.202007226
9. Abdollahiyan P, Oroojalian F, Mokhtarzadeh A, et al., 2020, Hydrogel-based 3D bioprinting for bone and cartilage tissue engineering. Biotechnol J, 15: e2000095.https://doi.org/10.1002/biot.202000095
10. Xiao S, Zhao T, Wang J, et al., 2019, Gelatin methacrylate (GelMA)-based hydrogels for cell transplantation: An effective strategy for tissue engineering. Stem Cell Rev Rep, 15: 664–679. https://doi.org/10.1007/s12015-019-09893-4
11. Nichol JW, Koshy ST, Bae H, et al., 2010, Cell-laden microengineered gelatin methacrylate hydrogels. Biomaterials, 31: 5536–5544. https://doi.org/10.1016/j.biomaterials.2010.03.064
12. Mei Q, Rao J, Bei HP, et al., 2021, 3D bioprinting photo-crosslinkable hydrogels for bone and cartilage repair. Int J Bioprint, 24: 367. https://doi.org/10.18063/ijb.v7i3.367
13. Zhang X, Li J, Ye P, et al., 2017, Coculture of mesenchymal stem cells and endothelial cells enhances host tissue integration and epidermis maturation through AKT activation in gelatin methacryloyl hydrogel-based skin model. Acta Biomater, 59: 317–326. https://doi.org/10.1016/j.actbio.2017.07.001
14. Sun X, Ma Z, Zhao X, et al., 2020, Three-dimensional bioprinting of multicell-laden scaffolds containing bone morphogenic protein-4 for promoting M2 macrophage polarization and accelerating bone defect repair in diabetes mellitus. Bioact Mater, 6: 757–769. https://doi.org/10.1016/j.bioactmat.2020.08.030
15. Zhang Y, Huang X, Yuan Y, 2017, MicroRNA-410 promotes chondrogenic differentiation of human bone marrow mesenchymal stem cells through down-regulating Wnt3a. Am J Transl Res, 9: 136–145.
16. Lara E, Araya M, Hill C, et al., 2021, Role of microRNA shuttled in small extracellular vesicles derived from mesenchymal stem/stromal cells for osteoarticular disease treatment. Front Immunol, 12: 768771. https://doi.org/10.3389/fimmu.2021.768771
17. Tian Y, Guo R, Shi B, et al., 2016, MicroRNA-30a promotes chondrogenic differentiation of mesenchymal stem cells through inhibiting Delta-like 4 expression. Life Sci, 148: 220–228. https://doi.org/10.1016/j.lfs.2016.02.031
18. Tornero-Esteban P, Hoyas JA, Villafuertes E, et al., 2014, Study of the role of miRNA in mesenchymal stem cells isolated from osteoarthritis patients. Rev Esp Cir Ortop Traumatol. 58: 138–43. https://doi.org/10.1016/j.recot.2013.12.004
19. Karlsen TA, Jakobsen RB, Mikkelsen TS, et al., 2014, MicroRNA-140 targets RALA and regulates chondrogenic differentiation of human mesenchymal stem cells by translational enhancement of SOX9 and ACAN. Stem Cells Dev, 23: 290–304. https://doi.org/10.1089/scd.2013.0209
20. Zhang H, Wang Y, Yang G, et al., 2019, MicroRNA-30a regulates chondrogenic differentiation of human bone marrow-derived mesenchymal stem cells through targeting Sox9. Exp Ther Med, 18: 4689–4697. https://doi.org/10.3892/etm.2019.8148
21. Yin Y, Ding L, Hou Y, et al., 2021, Correction to: Upregulating MicroRNA-410 or downregulating Wnt-11 increases osteoblasts and reduces osteoclasts to alleviate osteonecrosis of the femoral head. Nanoscale Res Lett, 9: 43. https://doi.org/10.1186/s11671-020-03465-z
22. Pan H, Dai H, Wang L, et al., 2020, MicroRNA-410-3p modulates chondrocyte apoptosis and inflammation by targeting high mobility group box 1 (HMGB1) in an osteoarthritis mouse model. BMC Musculoskelet Disord, 21: 486. https://doi.org/10.1186/s12891-020-03489-7
23. Kovács B, Vajda E, Nagy EE, 2019, Regulatory Effects and interactions of the Wnt and OPG-RANKL-RANK signaling at the bone-cartilage interface in osteoarthritis. Int J Mol Sci, 20: 4653. https://doi.org/10.3390/ijms20184653
24. Zhou Y, Zhao Z, Yan L, et al., 2021, MiR-485-3p promotes proliferation of osteoarthritis chondrocytes and inhibits apoptosis via Notch2 and the NF-κB pathway. Immunopharmacol Immunotoxicol, 43: 370–379. https://doi.org/10.1080/08923973.2021.1918150
25. Ma F, Li G, Yu Y, et al., 2019, MiR-33b-3p promotes chondrocyte proliferation and inhibits chondrocyte apoptosis and cartilage ECM degradation by targeting DNMT3A in osteoarthritis. Biochem Biophys Res Commun, 519: 430–437. https://doi.org10.1016/j.bbrc.2019.09.022
26. Liu J, Gao L, Zhan N, et al., 2020, Hypoxia induced ferritin light chain (FTL) promoted epithelia mesenchymal transition and chemoresistance of glioma. J Exp Clin Cancer Res, 39: 137. https://doi.org/10.1186/s13046-020-01641-8
27. Costantini M, Idaszek J, Szöke K, et al., 2016, 3D bioprinting of BM-MSCs-loaded ECM biomimetic hydrogels for in vitro neocartilage formation. Biofabrication, 8: 035002. https://doi.org/10.1088/1758-5090/8/3/035002
28. Zhang X, Chen Y, Zhang C, et al., 2021, Effects of icariin on the fracture healing in young and old rats and its mechanism. Pharm Biol, 59: 1245–1255. https://doi.org/10.1080/13880209.2021.1972121
29. Daly AC, Freeman FE, Gonzalez-Fernandez T, et al., 2017, 3D Bioprinting for Cartilage and osteochondral tissue engineering. Adv Healthc Mater, 6: 22. https://doi.org/10.1002/adhm.201700298
30. Liu Y, Peng L, Li L, et al., 2021, 3D-bioprinted BMSC-laden biomimetic multiphasic scaffolds for efficient repair of osteochondral defects in an osteoarthritic rat model. Biomaterials, 279: 121216. https://doi.org/10.1016/j.biomaterials.2021.121216
31. Zhang X, Liu Y, Zuo Q, et al., 2021, 3D bioprinting of biomimetic bilayered scaffold consisting of decellularized extracellular matrix and silk fibroin for osteochondral repair. Int J Bioprint, 7: 401. https://doi.org/10.18063/ijb.v7i4.401
32. Boere KW, Visser J, Seyednejad H, et al., 2014, Covalent attachment of a three-dimensionally printed thermoplast to a gelatin hydrogel for mechanically enhanced cartilage constructs. Acta Biomater, 10: 2602–2611. https://doi.org/10.1016/j.actbio.2014.02.041
33. Rothrauff B, Shimomura K, Gottardi R, et al., 2017, Anatomical region-dependent enhancement of 3-dimensional chondrogenic differentiation of human mesenchymal stem cells by soluble meniscus extracellular matrix. Acta Biomater, 49: 140–151. https://doi.org/10.1016/j.actbio.2016.11.046
34. Liu T, Weng W, Zhang Y, et al., 2020, Applications of gelatin methacryloyl (GelMA) hydrogels in microfluidic technique-assisted tissue engineering. Molecules, 25: 5305. https://doi.org/10.3390/molecules25225305
35. Jiang G, Li S, Yu K, et al., 2021, A 3D-printed PRP-GelMA hydrogel promotes osteochondral regeneration through M2 macrophage polarization in a rabbit model. Acta Biomater, 128: 150–162. https://doi.org/10.1016/j.actbio.2021.04.010
36. Suntornnond R, An J, Chua CK, 2017, Roles of support materials in 3D bioprinting-Present and future. Int J Bioprint, 3: 6. https://doi.org/10.18063/IJB.2017.01.006
37. Yang T, Zhang Q, Xie L, et al., 2021, HDPSC-laden GelMA microspheres fabricated using electrostatic microdroplet method for endodontic regeneration. Mater Sci Eng C Mater Biol Appl, 121: 111850. https://doi.org/10.1016/j.msec.2020.111850
38. Lin C, Wang Y, Huang Z, et al., 2021, Advances in filament structure of 3D bioprinted biodegradable bone repair scaffolds. Int J Bioprint, 7: 426. https://doi.org/10.18063/ijb.v7i4.426
39. Zhou L, Ramezani H, Sun M, et al., 2020, 3D printing of high-strength chitosan hydrogel scaffolds without any organic solvents. Biomater Sci, 8: 5020–5028. https://doi.org10.1039/d0bm00896f
40. Gao Q, Niu X, Shao L, et al., 2019, 3D printing of complex GelMA-based scaffolds with nanoclay. Biofabrication, 11: 035006. https://doi.org/10.1088/1758-5090/ab0cf6
41. Gao Q, Xie C, Wang P, et al., 2020, 3D printed multi-scale scaffolds with ultrafine fibers for providing excellent biocompatibility. Mater Sci Eng C Mater Biol Appl, 107: 110269. https://doi.org/10.1016/j.msec.2019.110269
42. Jensen C, Teng Y, 2020, Is it time to start transitioning from 2D to 3D cell culture. Front Mol Biosci, 7: 33. https://doi.org/10.3389/fmolb.2020.00033
43. Duval K, Grover H, Han LH, et al., 2017, Modeling physiological events in 2D vs. 3D cell culture. Physiol (Bethesda), 32: 266–277. https://doi.org/10.1152/physiol.00036.2016
44. Jiang D, Ge P, Wang L, et al., 2019, A novel electrochemical mast cell-based paper biosensor for the rapid detection of milk allergen casein. Biosens Bioelectron, 130: 299–306. https://doi.org/10.1016/j.bios.2019.01.050
45. Nazir F, Ashraf I, Iqbal M, et al., 2021, 6-deoxy-aminocellulose derivatives embedded soft gelatin methacryloyl (GelMA) hydrogels for improved wound healing applications: In vitro and in vivo studies. Int J Biol Macromol, 185: 419–433. https://doi.org/10.1016/j.ijbiomac
46. Mader M, Jérôme V, Freitag R, et al., 2018, Ultraporous, compressible, wettable polylactide/polycaprolactone sponges for tissue engineering. Biomacromolecules, 19: 1663–1673. https://doi.org/10.1021/acs.biomac.8b00434
47. Liang Y, Xu X, Li X, et al., 2020, Chondrocyte-targeted microrna delivery by engineered exosomes toward a cell-free osteoarthritis therapy. ACS Appl Mater Interfaces, 19: 36938–36947. https://doi.org/10.1021/acsami.0c10458
48. Yu A, Zhang T, Duan H, et al., 2017, MiR-124 contributes to M2 polarization of microglia and confers brain inflammatory protection via the C/EBP-α pathway in intracerebral hemorrhage. Immunol Lett, 182: 1–11. https://doi.org/10.1016/j.imlet.2016.12.003
49. Cazzanelli P, Wuertz-Kozak K, 2020, MicroRNAs in intervertebral disc degeneration, apoptosis, inflammation, and mechanobiology. Int J Mol Sci, 20: 3601. https://doi.org/10.3390/ijms21103601
50. Dong L, Pu Y, Zhang L, et al., 2018, Human umbilical
cord mesenchymal stem cell-derived extracellular vesicles promote lung adenocarcinoma growth by transferring miR- 410. Cell Death Dis, 9: 218.
https://doi.org/10.1038/s41419-018-0323-5
51. Wen R, Umeano AC, Essegian DJ, et al., Role of microRNA-410 in molecular oncology: A double edged sword. J Cell Biochem, 119: 8737–8742. https://doi.org/10.1002/jcb.27251
52. Li S, Liu Y, Zhang T, et al., 2022, A tetrahedral framework DNA-based bioswitchable miRNA inhibitor delivery system: Application to skin anti-aging. Adv Mater, 34: 2204287.https://doi.org/10.1002/adma.202204287
53. Cui X, Li J, Hartanto Y, et al., 2020, Advances in extrusion 3D bioprinting: A focus on multicomponent hydrogel-based bioinks. Adv Healthc Mater, 9: e1901648. https://doi.org/10.1002/adhm.201901648
54. Shao H, Liu A, Ke X, et al., 2017 3D robocasting magnesium-doped wollastonite/TCP bioceramic scaffolds with improved bone regeneration capacity in critical sized calvarial defects. J Mater Chem B, 5: 2941–2951. https://doi.org/10.1039/c7tb00217c
55. Hwangbo H, Kim W, Kim GH, 2021, Lotus-root-like microchanneled collagen scaffold. ACS Appl Mater Interfaces, 13: 12656–12667. https://doi.org/10.1021/acsami.0c14670
56. Lee HJ, Koo YW, Yeo M, et al., 2017, Recent cell printing systems for tissue engineering. Int J Bioprint, 3:4. https://doi.org/10.18063/IJB.2017.01.004
57. Li JW, Chen GJ, Xu XQ, et al., 2019, Advances of injectable hydrogel-based scaffolds for cartilage regeneration. Regen Biomater, 6: 129–140. https://doi.org/10.1093/rb/rbz022
58. Liu X, Hao M, Chen Z, 2021, 3D bioprinted neural tissue constructs for spinal cord injury repair. Biomaterials, 272: 120771. https://doi.org/10.1016/j.biomaterials.2021.120771
59. Li Z, Bi Y, Wu Q, et al., 2021, A composite scaffold of Wharton’s jelly and chondroitin sulphate loaded with human umbilical cord mesenchymal stem cells repairs articular cartilage defects in rat knee. J Mater Sci Mater Med, 29: 36. https://doi.org/10.1007/s10856-021-06506-w
60. Wang SJ, Jiang D, Zhang ZZ, et al., 2019, Biomimetic nanosilica-collagen scaffolds for in situ bone regeneration: Toward a cell-free, one-step surgery. Adv Mater, 31: e1904341. https://doi.org/10.1002/adma.201904341
61. Wang Y, Teng W, Zhang Z, et al., 2020, A trilogy antimicrobial strategy for multiple infections of orthopedic implants throughout their life cycle. Bioact Mater, 6: 1853–1866.https://doi.org/10.1016/j.bioactmat.2020.11.030
62. Zhu W, Cui H, Boualam B, et al., 2018, 3D bioprinting mesenchymal stem cell-laden construct with core-shell nanospheres for cartilage tissue engineering. Nanotechnology, 29: 185101. https://doi.org/10.1088/1361-6528/aaafa1
63. Li J, Li M, Chen X, et al., 2022, Repair of infected bone defect with clindamycin-tetrahedral DNA nanostructure complex-loaded 3D bioprinted hybrid scaffold. Chem Eng J, 435: 134855. https://doi.org/10.2139/ssrn.3967289