Antheraea pernyi silk fibroin bioinks for digital light processing 3D printing
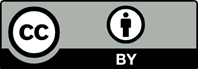
The application of three-dimensional (3D) bioprinting has increased in the biomedical field. The lack of bioinks with both biocompatibility and printability is still a problem to be solved. Silk fibroin materials have good biocompatibility and have a broad application prospect in the field of biomedical materials. At present, most research usually involves Bombyx mori silk fibroin (BSF). However, BSF has low cell adhesion. Compared with BSF, Antheraea pernyi silk fibroin (ASF) isolated from typical non-mulberry silk exhibits a unique arginine-glycine-aspartate (RGD) sequence with good cell adhesion enhancement. In this study, we developed a bioink based on ASF for digital light processing (DLP) 3D bioprinting. The ASF-based bioinks (ASF-MA) were produced by a methacryloylation process using methacrylic anhydride (MA) to achieve the properties of photopolymerization reaction. The ASF-MA hydrogel has mechanical properties, biocompatibility, and especially cell adhesion. Meanwhile, we found that the ASF-MA hydrogels promoted the adhesion, migration, and proliferation of S16 cells. Hence, the ASF-MA hydrogels had the potential applications in biomedical fields.
Yao X, Zou S, Fan S, et al., 2022, Bioinspired silk fibroin materials: From silk building blocks extraction and reconstruction to advanced biomedical applications. Mater Today Bio, 16: 100381. https://doi.org/10.1016/j.mtbio.2022.100381
Hu Y, Lee A, Chang S, et al., 2022, Biomaterial-induced conversion of quiescent cardiomyocytes into pacemaker cells in rats. Nat Biomed Eng, 6(4): 421–434. https://doi.org/10.1038/s41551-021-00812-y
Grigoryan B, Paulsen SJ, Corbett DC, et al., 2019, Multivascular networks and functional intravascular topologies within biocompatible hydrogels. Science, 364(6439): 458–464. https://doi.org/10.1126/science.aav9750
Lee A, Hudson AR, Shiwarski DJ, et al., 2019, 3D bioprinting of collagen to rebuild components of the human heart. Science, 365(6452): 482–487. https://doi.org/10.1126/science.aav9051
Yu C, Schimelman J, Wang P, et al., 2020, Photopolymerizable biomaterials and light-based 3D printing strategies for biomedical applications. Chem Rev, 120(19): 10695–10743. https://doi.org/10.1021/acs.chemrev.9b00810
Unagolla JM, Jayasuriya AC, 2020, Hydrogel-based 3D bioprinting: A comprehensive review on cell-laden hydrogels, bioink formulations, and future perspectives. Appl Mater Today, 18: 100479. https://doi.org/10.1016/j.apmt.2019.100479
Kim SH, Hong H, Ajiteru O, et al., 2021, 3D bioprinted silk fibroin hydrogels for tissue engineering. Nat Protoc, 16(12): 5484–5532. https://doi.org/10.1038/s41596-021-00622-1
Hong H, Seo YB, Kim DY, et al., 2020, Digital light processing 3D printed silk fibroin hydrogel for cartilage tissue engineering. Biomaterials, 232:119679. https://doi.org/10.1016/j.biomaterials.2019.119679
Lee YJ, Lee JS, Ajiteru O, et al., 2022, Biocompatible fluorescent silk fibroin bioink for digital light processing 3D printing. Int J Biol Macromol, 213:317–327. https://doi.org/10.1016/j.ijbiomac.2022.05.123
Wu X, Zhou M, Jiang F, et al., 2021, Marginal sealing around integral bilayer scaffolds for repairing osteochondral defects based on photocurable silk hydrogels. Bioact Mater, 6(11): 3976–3986. https://doi.org/10.1016/j.bioactmat.2021.04.005
Zou S, Yao X, Shao H, et al., 2022, Nonmulberry silk fibroin-based biomaterials: Impact on cell behavior regulation and tissue regeneration. Acta Biomater, 153: 68–84. https://doi.org/10.1016/j.actbio.2022.09.021
Tang X, Chen X, Zhang S, et al., 2021, Silk-inspired in situ hydrogel with anti-tumor immunity enhanced photodynamic therapy for melanoma and infected wound healing. Adv Funct Mater, 31(17): 2101320. https://doi.org/10.1002/adfm.202101320
Naskar D, Sapru S, Ghosh AK, et al., 2021, Nonmulberry silk proteins: Multipurpose ingredient in bio-functional assembly. Biomed Mater, 16(6): 062002. https://doi.org/10.1088/1748-605X/ac20a0
Ma Y, Duan L, Sun J, et al., 2022, Oral nanotherapeutics based on Antheraea pernyi silk fibroin for synergistic treatment of ulcerative colitis. Biomaterials, 282: 121410. https://doi.org/10.1016/j.biomaterials.2022.121410
Kim SH, Yeon YK, Lee JM, et al., 2018, Precisely printable and biocompatible silk fibroin bioink for digital light processing 3D printing. Nat Commun, 9(1): 1620. https://doi.org/10.1038/s41467-018-03759-y
Li X, Zhang Q, Ye D, et al., 2017, Fabrication and characterization of electrospun PCL/Antheraea pernyi silk fibroin nanofibrous scaffolds. Polym Eng Sci, 57(2): 206–213. https://doi.org/10.1002/pen.24402
Lee K, Kweon H, Yeo J, et al., 2011, Characterization of tyrosine-rich Antheraea pernyi silk fibroin hydrolysate. Int J Biol Macromol, 48(1): 223–226. https://doi.org/10.1016/j.ijbiomac.2010.09.020
Guan J, Zhu W, Liu B, et al., 2017, Comparing the microstructure and mechanical properties of Bombyx mori and Antheraea pernyi cocoon composites. Acta Biomater, 47: 60–70. https://doi.org/10.1016/j.actbio.2016.09.042
Yang K, Guan J, Numata K, et al., 2019, Integrating tough Antheraea pernyi silk and strong carbon fibres for impact-critical structural composites. Nat Commun, 10(1): 3786. https://doi.org/10.1038/s41467-019-11520-2
Wang J, Chen Y, Zhou G, et al., 2019, Polydopamine-coated Antheraea pernyi (A. pernyi) silk fibroin films promote cell adhesion and wound healing in skin tissue repair. ACS Appl Mater Interfaces, 11(38): 34736–34743. https://doi.org/10.1021/acsami.9b12643
Wang J, Yin Z, Xue X, et al., 2016, Natural non-mulberry silk nanoparticles for potential-controlled drug release. Int J Mol Sci, 17(12): 2012. https://doi.org/10.3390/ijms17122012
Cui B, Zhang C, Gan B, et al., 2020, Collagen-tussah silk fibroin hybrid scaffolds loaded with bone mesenchymal stem cells promote skin wound repair in rats. Mater Sci Eng C, 109: 110611. https://doi.org/10.1016/j.msec.2019.110611
Zou S, Wang X, Fan S, et al., 2019, Fabrication and characterization of regenerated Antheraea pernyi silk fibroin scaffolds for Schwann cell culturing. Eur Polym J, 117: 123–133. https://doi.org/10.1016/j.eurpolymj.2019.04.056
Yan S, Zhao C, Wu X, et al., 2010, Gelation behavior of Antheraea pernyi silk fibroin. Sci China Chem, 53(3): 535–541. https://doi.org/10.1007/s11426-010-0093-0
Lee BH, Lum N, Seow LY, et al., 2016, Synthesis and characterization of types A and B gelatin methacryloyl for bioink applications. Materials, 9(10): 797. https://doi.org/10.3390/ma9100797
Carbonaro M, Nucara A, 2010, Secondary structure of food proteins by Fourier transform spectroscopy in the mid-infrared region. Amino Acids, 38(3): 679–690. https://doi.org/10.1007/s00726-009-0274-3
Taddei P, Tsukada M,Freddi G, 2013, Affinity of protein fibres towards sulfation. J Raman Spectrosc, 44(2): 190–197. https://doi.org/10.1002/jrs.4168
Shirahama H, Lee BH, Tan LP, et al., 2016, Precise tuning of facile one-pot gelatin methacryloyl (GelMA) synthesis. Sci Rep, 6(1): 31036. https://doi.org/10.1038/srep31036
Kumar M, Gupta P, Bhattacharjee S, et al., 2018, Immunomodulatory injectable silk hydrogels maintaining functional islets and promoting anti-inflammatory M2 macrophage polarization. Biomaterials, 187: 1–17.
https://doi.org/10.1016/j.biomaterials.2018.09.037
Li XF, Zhang J, Feng YF, et al., 2018, Tuning the structure and performance of silk biomaterials by combining mulberry and non-mulberry silk fibroin. Polym Degrad Stab, 147: 57–63. https://doi.org/10.1016/j.polymdegradstab.2017.11.013
Slaughter BV, Khurshid SS, Fisher OZ, et al., 2009, Hydrogels in regenerative medicine. Adv Mater, 21(32–33): 3307–3329. https://doi.org/10.1002/adma.200802106
Xiao WQ, He JK, Nichol JW, et al., 2011, Synthesis and characterization of photocrosslinkable gelatin and silk fibroin interpenetrating polymer network hydrogels. Acta Biomater, 7(6): 2384–2393. https://doi.org/10.1016/j.actbio.2011.01.016
Dadras Chomachayi M, Solouk A, Mirzadeh H, 2019, Improvement of the electrospinnability of silk fibroin solution by atmospheric pressure plasma treatment. Fibers Polym, 20(8): 1594–1600. https://doi.org/10.1007/s12221-019-9015-8
Gu Y, Ji Y, Zhao Y, et al., 2012, The influence of substrate stiffness on the behavior and functions of Schwann cells in culture. Biomaterials, 33(28): 6672–6681. https://doi.org/10.1016/j.biomaterials.2012.06.006
Xu Y, Gu Y, Cai F, et al., 2020, Metabolism balance regulation via antagonist-functionalized injectable microsphere for nucleus pulposus regeneration. Adv Funct Mater, 30(52): 2006333. https://doi.org/10.1002/adfm.202006333
Sun J, Li J, Huan Z, et al., 2023, Mesenchymal stem cell-laden composite β cell porous microgel for diabetes treatment. Adv Funct Mater, 2211897. https://doi.org/10.1002/adfm.202211897
Hu Y, Zhang Q, You R, et al., 2012, The relationship between secondary structure and biodegradation behavior of silk fibroin scaffolds. Adv Mater Sci Eng, 2012: 185905. https://doi.org/10.1155/2012/185905
Tao J, Zhang J, Du T, et al., 2019, Rapid 3D printing of functional nanoparticle-enhanced conduits for effective nerve repair. Acta Biomater, 90: 49–59. https://doi.org/10.1016/j.actbio.2019.03.047
Xu X, Tao J, Wang S, et al., 2019, 3D printing of nerve conduits with nanoparticle-encapsulated RGFP966. Appl Mater Today, 16: 247–256. https://doi.org/10.1016/j.apmt.2019.05.014
Dendukuri D, Pregibon DC, Collins J, et al., 2006, Continuous-flow lithography for high-throughput microparticle synthesis. Nat Mater, 5(5): 365–369. https://doi.org/10.1038/nmat1617
DUrso D, Ehrhardt P, Müller HW, 1999, Peripheral myelin protein 22 and protein zero: A novel association in peripheral nervous system myelin. J Neurosci, 19(9): 3396. https://doi.org/10.1523/JNEUROSCI.19-09-03396.1999
Sasagasako N, Toda K, Hollis M, et al., 1996, Myelin gene expression in immortalized schwann cells: Relationship to cell density and proliferation. J Neurochem, 66(4): 1432– 1439. https://doi.org/10.1046/j.1471-4159.1996.66041432.x
Sasagasako N, Ohno M,Quarles RH, 1999, Evidence for regulation of myelin protein synthesis by contact between adjacent schwann cell plasma membranes. Dev Neurosci, 21(6): 417–422. https://doi.org/10.1159/000017409
Li G, Zhao X, Zhang L, et al., 2020, Anisotropic ridge/groove microstructure for regulating morphology and biological function of Schwann cells. Appl Mater Today, 18: 100468. https://doi.org/10.1016/j.apmt.2019.100468
Tylek T, Blum C, Hrynevich A, et al., 2020, Precisely defined fiber scaffolds with 40 μm porosity induce elongation driven M2-like polarization of human macrophages. Biofabrication, 12(2): 025007. https://doi.org/10.1088/1758-5090/ab5f4e
Dai Y, Li X, Wu R, et al., 2018, Macrophages of different phenotypes influence the migration of BMSCs in PLGA scaffolds with different pore size. Biotechnol J, 13(1): 1700297. https://doi.org/10.1002/biot.201700297
Song E, Yeon Kim S, Chun T, et al., 2006, Collagen scaffolds derived from a marine source and their biocompatibility. Biomaterials, 27(15): 2951–2961. https://doi.org/10.1016/j.biomaterials.2006.01.015
Altman GH, Diaz F, Jakuba C, et al., 2003, Silk-based biomaterials. Biomaterials, 24(3): 401–416. https://doi.org/10.1016/S0142-9612(02)00353-8
Xie H, Gu Z, Li C, et al., 2016, A novel bioceramic scaffold integrating silk fibroin in calcium polyphosphate for bone tissue-engineering. Ceram Int, 42(2, Part A): 2386–2392. https://doi.org/10.1016/j.ceramint.2015.10.036
You R, Xu Y, Liu Y, et al., 2015, Comparison of the in vitro and in vivo degradations of silk fibroin scaffolds from mulberry and nonmulberry silkworms. Biomed Mater, 10(1): 015003. https://doi.org/10.1088/1748-6041/10/1/015003
Guo C, Zhang J, Jordan JS, et al., 2018, Structural comparison of various silkworm silks: An insight into the structure– property relationship. Biomacromolecules, 19(3): 906–917. https://doi.org/10.1021/acs.biomac.7b01687
Singh D, Harding AJ, Albadawi E, et al., 2018, Additive manufactured biodegradable poly(glycerol sebacate methacrylate) nerve guidance conduits. Acta Biomater, 78: 48–63. https://doi.org/10.1016/j.actbio.2018.07.055
Guimarães CF, Gasperini L, Marques AP, et al., 2020, The stiffness of living tissues and its implications for tissue engineering. Nat Rev Mater, 5(5): 351–370. https://doi.org/10.1038/s41578-019-0169-1
Guvendiren M, Molde J, Soares RMD, et al., 2016, Designing biomaterials for 3D printing. ACS Biomater Sci Eng, 2(10): 1679–1693. https://doi.org/10.1021/acsbiomaterials.6b00121
Kim S, Kawai T, Wang D, et al., 2016, Engineering a dual-layer chitosan–lactide hydrogel to create endothelial cell aggregate-induced microvascular networks in vitro and increase blood perfusion in vivo. ACS Appl Mater Interfaces, 8(30): 19245–19255. https://doi.org/10.1021/acsami.6b04431
Pandit V, Zuidema JM, Venuto KN, et al., 2013, Evaluation of multifunctional polysaccharide hydrogels with varying stiffness for bone tissue engineering. Tissue Eng Part A, 19(21–22): 2452–2463. https://doi.org/10.1089/ten.tea.2012.0644
Rosso G, Liashkovich I, Young P, et al., 2017, Schwann cells and neurite outgrowth from embryonic dorsal root ganglions are highly mechanosensitive. Nanomedicine, 13(2): 493–501. https://doi.org/10.1016/j.nano.2016.06.011
Wu Y-X, Ma H, Wang J-L, et al., 2021, Production of chitosan scaffolds by lyophilization or electrospinning: Which is better for peripheral nerve regeneration? Neural Regen Res, 16(6): 1093–1098. https://doi.org/10.4103/1673-5374.300463
Naghilou A, Pöttschacher L, Millesi F, et al., 2020, Correlating the secondary protein structure of natural spider silk with its guiding properties for Schwann cells. Mater Sci Eng C, 116: 111219. https://doi.org/10.1016/j.msec.2020.111219