Laser bioprinting of human iPSC-derived neural stem cells and neurons: Effect on cell survival, multipotency, differentiation, and neuronal activity
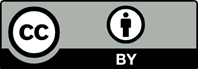
Generation of human neuronal networks by three-dimensional (3D) bioprinting is promising for drug testing and hopefully will allow for the understanding of cellular mechanisms in brain tissue. The application of neural cells derived from human induced-pluripotent stem cells (hiPSCs) is an obvious choice, since hiPSCs provide access to cells unlimited in number and cell types that could be generated by differentiation. The questions in this regard include which neuronal differentiation stage is optimal for printing of such networks, and to what extent the addition of other cell types, especially astrocytes, supports network formation. These aspects are the focus of the present study, in which we applied a laser-based bioprinting technique and compared hiPSC-derived neural stem cells (NSCs) with neuronal differentiated NSCs, with and without the inclusion of co-printed astrocytes. In this study, we investigated in detail the effects of cell types, printed droplet size, and duration of differentiation before and after printing on viability, as well as proliferation, stemness, differentiation potential, formation of dendritic extensions and synapses, and functionality of the generated neuronal networks. We found a significant dependence of cell viability after dissociation on differentiation stage, but no impact of the printing process. Moreover, we observed a dependence of the abundance of neuronal dendrites on droplet size, a marked difference between printed cells and normal cell culture in terms of further differentiation of the cells, especially differentiation into astrocytes, as well as neuronal network formation and activity. Notably, there was a clear effect of admixed astrocytes on NSCs but not on neurons.
1. WHO, 2006, Neurological Disorders: Public Health Challenges, World Health Organization, Geneva, Switzerland, 204, table A.4.7.
2. Zhuang P, Sun AX, An J, et al., 2018, 3D neural tissue models: From spheroids to bioprinting. Biomaterials, 154: 113–133. https://doi.org/10.1016/j.biomaterials.2017.10.002
3. Turunen S, Joki T, Hiltunen ML, et al., 2017, Direct laser writing of tubular microtowers for 3D culture of human pluripotent stem cell-derived neuronal cells. ACS Appl Mater Interfaces, 9:25717–25730. https://doi.org/10.1021/acsami.7b05536
4. Cantley WL, Du C, Lomoio S, et al., 2018, Functional and sustainable 3D human neural network models from pluripotent stem cells. ACS Biomater Sci Eng, 4:4278–4288. https://doi.org/10.1021/acsbiomaterials.8b00622
5. Jakobsson A, Ottosson M, Zalis MC, et al., 2017, Three-dimensional functional human neuronal networks in uncompressed low-density electrospun fiber scaffolds. Nanomed Nanotechnol Biol Med, 13:1563–1573. https://doi.org/10.1016/j.nano.2016.12.023
6. Koroleva A, Deiwick A, El-Tamer A, et al., 2021, In vitro development of human iPSC-derived functional neuronal networks on laser-fabricated 3D scaffolds. ACS Appl Mater Interfaces, 13:7839–7853. https://doi.org/10.1021/acsami.0c16616
7. Lancaster MA, Renner M, Martin CA, et al., 2013, Cerebral organoids model human brain development and microcephaly. Nature, 501:373–379. https://doi.org/10.1038/nature12517
8. Camp JG, Badsha F, Florio M, et al., 2015, Human cerebral organoids recapitulate gene expression programs of fetal neocortex development. Proc Natl Acad Sci USA, 112:15672– 15677. https://doi.org/10.1073/pnas.1520760112
9. Quadrato G, Brown J, Arlotta P, 2016, The promises and challenges of human brain organoids as models of neuropsychiatric disease. Nat Med, 22:1220–1228. https://doi.org/10.1038/nm.4214
10. Birey F, Andersen J, Makinson CD, et al., 2017, Assembly of functionally integrated human forebrain spheroids. Nature, 545:54–59. https://doi.org/10.1038/nature22330
11. Hofrichter M, Nimtz L, Tigges J, et al., 2017, Comparative performance analysis of human iPSC-derived and primary neural progenitor cells (NPC) grown as neurospheres in vitro. Stem Cell Res, 25:72–82. https://doi.org/10.1016/j.scr.2017.10.013
12. Takahashi K, Tanabe K, Ohnuki M, et al., 2007, Induction of pluripotent stem cells from adult human fibroblasts by defined factors. Cell, 131:861–872. https://doi.org/10.1016/j.cell.2007.11.019
13. Haase A, Olmer R, Schwanke K, et al., 2009, Generation of induced pluripotent stem cells from human cord blood. Cell Stem Cell, 5:434–441. https://doi.org/10.1016/j.stem.2009.08.021
14. Shi Y, Kirwan P, Livesey FJ, 2012, Directed differentiation of human pluripotent stem cells to cerebral cortex neurons and neural networks. Nat Protoc, 7:1836–1846. https://doi.org/10.1038/nprot.2012.116
15. Shi, Y, Inoue H, Wu JC, et al., 2017, Induced pluripotent stem cell technology: A decade of progress. Nat Rev Drug Discov, 16:115–130. https://doi.org/10.1038/nrd.2016.245
16. Canfield SG, Stebbins MJ, Faubion MG, et al., 2019, An isogenic neurovascular unit model comprised of human induced pluripotent stem cell-derived brain microvascular endothelial cells, pericytes, astrocytes, and neurons. Fluids Barriers CNS, 16:1–12. https://doi.org/10.1186/s12987-019-0145-6
17. Marton RM, Miura Y, Sloan SA, et al., 2019, Differentiation and maturation of oligodendrocytes in human three-dimensional neural cultures. Nat Neurosci, 22:484–491. https://doi.org/10.1038/s41593-018-0316-9
18. Xu T, Gregory CA, Molnar P, et al., 2006, Viability and electrophysiology of neural cell structures generated by the inkjet printing method. Biomaterials, 27:3580–3588. https://doi.org/10.1016/j.biomaterials.2006.01.048
19. Lee W, Pinckney J, Lee V, et al., 2009, Three-dimensional bioprinting of rat embryonic neural cells. NeuroReport, 20:798–803. https://doi.org/10.1097/WNR.0b013e32832b8be4
20. Lorber B, Hsiao WK, Hutchings IM, et al., 2014, Adult rat retinal ganglion cells and glia can be printed by piezoelectric inkjet printing. Biofabrication, 6:015001. https://doi.org/10.1088/1758-5082/6/1/015001
21. Lozano R, Stevens L, Thompson BC, et al., 2015, 3D printing of layered brain-like structures using peptide modified gellan gum substrates. Biomaterials, 67:264–273. https://doi.org/10.1016/j.biomaterials.2015.07.022
22. Kador KE, Venugopalan P, Malek MF, et al., 2016, Control of retinal ganglion cell positioning and neurite growth: Combining 3D printing with radial electrospun scaffolds. Tissue Eng Part A, 22:286–294. https://doi.org/10.1089/ten.TEA.2015.0373
23. Curley JL, Sklare SC, Bowser DA, et al., 2016, Isolated node engineering of neuronal systems using laser direct write. Biofabrication, 8:015013. https://doi.org/10.1088/1758-5090/8/1/015013
24. Song Y, Su X, Firouzian KF, et al., 2020, Engineering of brain-like tissue constructs via 3D cell-printing technology, Biofabrication, 12:035016. https://doi.org/10.1088/1758-5090/ab7d76
25. Roversi K, Ebrahimi Orimi H, Falchetti M, et al., 2021, Bioprinting of adult dorsal root ganglion (DRG) neurons using laser-induced side transfer (LIST). Micromachines, 12, 865. https://doi.org/10.3390/mi12080865
26. Lee, YB, Polio S, Lee W, et al., 2010, Bio-printing of collagen and VEGF-releasing fibrin gel scaffolds for neural stem cell culture. Exp Neurol, 223:645–652. https://doi.org/10.1016/j.expneurol.2010.02.014
27. Hsieh FY, Lin HH, Hsu SH, 2015, 3D bioprinting of neural stem cell-laden thermoresponsive biodegradable polyurethane hydrogel and potential in central nervous system repair. Biomaterials, 71:48–57. https://doi.org/10.1016/j.biomaterials.2015.08.028
28. Joung D, Truong V, Neitzke CC, et al., 2018, 3D printed stem-cell derived neural progenitors generate spinal cord scaffolds. Adv Funct Mater, 28:1801850. https://doi.org/10.1002/adfm.201801850
29. De la Vega L, Rosas Gómez AD, Abelseth E, et al., 2018, 3D bioprinting human induced pluripotent stem cell-derived neural tissues using a novel lab-on-a-printer technology. Appl Sci, 8:2414. https://doi.org/10.3390/app8122414
30. Salaris F, Colosi C, Brighi C, et al., 2019, 3D bioprinted human cortical neural constructs derived from induced pluripotent stem cells. J Clin Med, 8:1595. https://doi.org/10.3390/jcm8101595
31. Fantini V, Bordoni M, Scocozza F, et al 2019, Bioink composition and printing parameters for 3D modeling neural tissue. Cells, 8:830. https://doi.org/10.3390/cells8080830
32. Sharma R, Smits IPM, De La Vega L, et al., 2020, 3D bioprinting pluripotent stem cell derived neural tissues using a novel fibrin bioink containing drug releasing microspheres. Front Bioeng Biotechnol, 8:57. https://doi.org/10.3389/fbioe.2020.00057
33. Zhou L, Wolfes AC, Li Y, et al., 2020, 3D lipid‐bilayer‐supported 3D printing of human cerebral cortex cells reveals developmental interactions. Adv Mater, 32:e2002183. https://doi.org/10.1002/adma.202002183
34. Gu Q, Tomaskovic-Crook E, Wallace GG, et al., 2017, 3D bioprinting human induced pluripotent stem cell constructs for in situ cell proliferation and successive multilineage differentiation. Adv Healthcare Mater, 6:1700175. https://doi.org/10.1002/adhm.201700175
35. Gu Q, Tomaskovic-Crook E, Lozano R, et al., 2016, Functional 3D neural mini‐tissues from printed gel‐based bioink and human neural stem cells. Adv Healthcare Mater, 5:1429–1438. https://doi.org/10.1002/adhm.201600095
36. England S, Rajaram A, Schreyer DJ, et al., 2017, Bioprinted fibrin-factor XIII-hyaluronate hydrogel scaffolds encapsulated Schwann cells and their in vitro characterization for use in nerve regeneration. Bioprinting, 5:1–9. https://doi.org/10.1016/j.bprint.2016.12.001
37. Ning L, Sun H, Lelong T, et al., 2018, 3D bioprinting of scaffolds with living Schwann cells for potential nerve tissue engineering applications. Biofabrication, 10:035014. https://doi.org/10.1088/1758-5090/aacd30
38. Li X, Wang X, Wang X, et al., 2018, 3D bioprinted rat Schwann cell-laden structures with shape flexibility and enhanced nerve growth factor expression. Biotech, 8:342. https://doi.org/10.1007/s13205-018-1341-9
39. Haring AP, Thompson EG, Tong Y, et al., 2019, Process-and bio-inspired hydrogels for 3D bioprinting of soft free-standing neural and glial tissues. Biofabrication, 11:025009. https://doi.org/10.1088/1758-5090/ab02c9
40. Wu Z, Li Q, Xie S, et al., 2020, In vitro and in vivo biocompatibility evaluation of a 3D bioprinted gelatin-sodium alginate/rat Schwann-cell scaffold. Mater Sci Eng C Mater Biol Appl, 109:110530. https://doi.org/10.1016/j.msec.2019.110530
41. Tse C, Whiteley R, Yu T, et al., 2016, Inkjet printing Schwann cells and neuronal analogue NG108-15 cells. Biofabrication, 8:015017. https://doi.org/10.1088/1758-5090/8/1/015017
42. Patz TM, Doraiswamy A, Narayan RJ, et al., 2006, Three-dimensional direct writing of B35 neuronal cells. J Biomed Mater Res B, 78:124–130. https://doi.org/10.1002/jbm.b.30473
43. Antill-O’Brien N, Bourke J, O’Connell CD, 2019, Layer-by-layer: The case for 3D bioprinting neurons to create patient-specific epilepsy models. Materials, 12:3218. https://doi.org/10.3390/ma12193218
44. Salaris F, Rosa A, 2019, Construction of 3D in vitro models by bioprinting human pluripotent stem cells: Challenges and opportunities. Brain Res, 1723:146393. https://doi.org/10.1016/j.brainres.2019.146393
45. Koch L, Deiwick A, Franke A, et al., 2018, Laser bioprinting of human induced pluripotent stem cells—The effect of printing and biomaterials on cell survival, pluripotency, and differentiation. Biofabrication, 10:035005. https://doi.org/10.1088/1758-5090/aab981
46. Koch L, Brandt O, Deiwick A, et al., 2016, Laser-assisted bioprinting at different wavelengths and pulse durations with a metal dynamic release layer: A parametric study. Int J Bioprint, 3:42–53. https://doi.org/10.18063/IJB.2017.01.001
47. Unger C, Gruene M, Koch L, et al., 2011, Time-resolved imaging of hydrogel printing via laser-induced forward transfer. Appl Phys A, 103:271–277. https://doi.org/10.1007/s00339-010-6030-4
48. Gruene M, Deiwick A, Koch L, et al., 2011, Laser printing of stem cells for biofabrication of scaffold-free autologous grafts. Tissue Eng Part C Methods, 17:79. https://doi.org/10.1089/ten.tec.2010.0359
49. Orlandi JG, Fernández-García S, Comella-Bolla A, et al., 2017, NETCAL: An interactive platform for large-scale, NETwork and population dynamics analysis of CALcium imaging recordings (Version 7.0.0 Open Beta), Zenodo. https://doi.org/10.5281/ZENODO.1119026
50. Lütcke H, Gerhard F, Zenke F, et al., 2013, Inference of neuronal network spike dynamics and topology from calcium imaging data. Front Neural Circuits, 7:201. https://doi.org/10.3389/fncir.2013.00201
51. Ludl AA, Soriano J, 2020, Impact of physical obstacles on the structural and effective connectivity of in silico neuronal circuits. Front Comput Neurosci, 14:77. https://doi.org/10.3389/fncom.2020.00077
52. Stetter O, Battaglia D, Soriano J, et al., 2012, Model-free reconstruction of excitatory neuronal connectivity from calcium imaging signals. PLoS Comput Biol, 8:e1002653. https://doi.org/10.1371/journal.pcbi.1002653
53. Orlandi JG, Stetter O, Soriano J, et al., 2014, Transfer entropy reconstruction and labeling of neuronal connections from simulated calcium imaging. PLoS One, 9:e98842. https://doi.org/10.1371/journal.pone.0098842
54. Tibau E, Ludl AA, Rudiger S, et al., 2020, Neuronal spatial arrangement shapes effective connectivity traits of in vitro cortical networks. IEEE Trans Netw Sci Eng, 7:435–448. https://doi.org/10.1109/TNSE.2018.2862919
55. Rubinov M, Sporns O, 2010, Complex network measures of brain connectivity: uses and interpretations. Neuroimage, 52:1059–1069. https://doi.org/10.1016/j.neuroimage.2009.10.003
56. Latora V, Marchiori M, 2001, Efficient behavior of small-world networks. Phys Rev Lett, 87:198701. https://doi.org/10.1103/PhysRevLett.87.198701
57. Blondel VD, Guillaume JL, Lambiotte R, et al., 2009, Fast unfolding pf communities in large networks. J Stat Mech, P10008:1–12. https://doi.org/10.1088/1742-5468/2008/10/P10008
58. Knight VB, Serrano EE, 2017, Post-translational tubulin modifications in human astrocyte cultures. Neurochem Res, 42(9):2566–2576. https://doi.org/10.1007/s11064-017-2290-0
59. Verwer RW, Sluiter AA, Balesar RA, et al., 2007, Mature astrocytes in the adult human neocortex express the early neuronal marker doublecortin. Brain, 130(Pt 12):3321– 3335. https://doi.org/10.1093/brain/awm264
60. Sahara S, Yanagawa Y, O’Leary DDM, et al., 2012, The fraction of cortical GABAergic neurons is constant from near the start of cortical neurogenesis to adulthood. J Neurosci, 32:4755–4761. https://doi.org/10.1523/JNEUROSCI.6412-11.2012
61. Hanada T, 2020, Ionotropic glutamate receptors in epilepsy: A review focusing on AMPA and NMDA receptors. Biomolecules, 10:464. https://doi.org/10.3390/biom10030464
62. Berridge MJ, Lipp P, Bootman MD, 2000, The versatility and universality of calcium signaling. Nat Rev Mol Cell Biol, 1:11–21. https://doi.org/10.1038/35036035
63. Grienberger C, Konnerth A, 2012, Imaging calcium in neurons. Neuron, 73:862. https://doi.org/10.1016/j.neuron.2012.02.011
64. Klapper SD, Garg P, Dagar S, et al., 2019, Astrocyte lineage cells are essential for functional neuronal differentiation and synapse maturation in human iPSC-derived neural networks. Glia, 67:1893–1909. https://doi.org/10.1002/glia.23666
65. Farhy-Tselnicker I, Allen NJ, 2018, Astrocytes, neurons, synapses: A tripartite view on cortical circuit development. Neural Dev, 13:7. https://doi.org/10.1186/s13064-018-0104-y