FeS2-incorporated 3D PCL scaffold improves new bone formation and neovascularization in a rat calvarial defect model
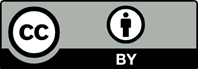
Three-dimensional (3D) scaffolds composed of various biomaterials, including metals, ceramics, and synthetic polymers, have been widely used to regenerate bone defects. However, these materials possess clear downsides, which prevent bone regeneration. Therefore, composite scaffolds have been developed to compensate these disadvantages and achieve synergetic effects. In this study, a naturally occurring biomineral, FeS2, was incorporated in PCL scaffolds to enhance the mechanical properties, which would in turn influence the biological characteristics. The composite scaffolds consisting of different weight fractions of FeS2 were 3D printed and compared to pure PCL scaffold. The surface roughness (5.77-fold) and the compressive strength (3.38-fold) of the PCL scaffold was remarkably enhanced in a dose-dependent manner. The in vivo results showed that the group with PCL/FeS2 scaffold implanted had increased neovascularization and bone formation (2.9-fold). These results demonstrated that the FeS2 incorporated PCL scaffold might be an effective bioimplant for bone tissue regeneration.
1. Boskey AL, 2013, Bone composition: Relationship to bone fragility and antiosteoporotic drug effects. Bonekey Rep, 2: 447.
2. Datta H, Ng W, Walker J, et al., 2008, The cell biology of bone metabolism. J Clin Pathol, 61(5): 577–587.
3. Lanyon L, 1992, The success and failure of the adaptive response to functional load-bearing in averting bone fracture. Bone, 13: S17–S21.
4. Phillips A, 2005, Overview of the fracture healing cascade. Injury, 36(3): S5–S7.
5. Baker CE, Moore-Lotridge SN, Hysong AA, et al., 2018, Bone fracture acute phase response—A unifying theory of fracture repair: Clinical and scientific implications. Clin Rev Bone Miner Metab, 16(4): 142–158.
6. Damien CJ, Parsons JR, 1991, Bone graft and bone graft substitutes: A review of current technology and applications. J Appl Biomater, 2(3): 187–208.
7. Puelacher W, Vacanti J, Ferraro N, et al., 1996, Femoral shaft reconstruction using tissue-engineered growth of bone. Int J Oral Maxillofac Surg, 25(3): 223–228.
8. LaPrade RF, Botker JC, 2004, Donor-site morbidity after osteochondral autograft transfer procedures. Arthrosc J Arthrosc Relat Surg, 20(7): e69–e73.
9. Laurencin C, Khan Y, El-Amin SF, 2006, Bone graft substitutes. Expert Rev Med Devices, 3(1): 49–57.
10. Vacanti JP, Langer R. 1999, Tissue engineering: The design and fabrication of living replacement devices for surgical reconstruction and transplantation. Lancet, 354: S32–S34.
11. Chang H-I, Wang Y, 2011, Regenerative Medicine and Tissue Engineering—Cells and Biomaterials, Eberli D editor, UK: InTech.
12. Bose S, Roy M, Bandyopadhyay A, 2012, Recent advances in bone tissue engineering scaffolds. Trends Biotechnol, 30(10): 546–554.
13. Saunders RE, Derby B. 2014, Inkjet printing biomaterials for tissue engineering: Bioprinting. Int Mater Rev, 59(8): 430–448.
14. Gudapati H, Dey M, Ozbolat I, 2016, A comprehensive review on droplet-based bioprinting: Past, present and future. Biomaterials, 102: 20–42.
15. Melchels FP, Feijen J, Grijpma DW, 2010, A review on stereolithography and its applications in biomedical engineering. Biomaterials, 31(24): 6121–6130.
16. Skoog SA, Goering PL, Narayan RJ, 2014, Stereolithography in tissue engineering. J Mater Sci Mater Med, 25(3): 845–856.
17. Ozbolat IT, Hospodiuk M, 2016, Current advances and future perspectives in extrusion-based bioprinting. Biomaterials, 76: 321–343.
18. Paxton N, Smolan W, Böck T, et al., 2017, Proposal to assess printability of bioinks for extrusion-based bioprinting and evaluation of rheological properties governing bioprintability. Biofabrication, 9(4): 044107.
19. Li X, Liu B, Pei B, et al., 2020, Inkjet bioprinting of biomaterials. Chem Rev, 120(19): 10793–10833.
20. Ng WL, Lee JM, Zhou M, et al., 2020, Vat polymerization based bioprinting—Process, materials, applications and regulatory challenges. Biofabrication, 12(2): 022001.
21. Jiang T, Munguia-Lopez JG, Flores-Torres S, et al., 2019, Extrusion bioprinting of soft materials: An emerging technique for biological model fabrication. Appl Phys Rev, 6(1): 011310.
22. Du X, Fu S, Zhu Y, 2018, 3D printing of ceramic-based scaffolds for bone tissue engineering: An overview. J Mater Chem B, 6(27): 4397–4412.
23. Liu X, Ma PX, 2004, Polymeric scaffolds for bone tissue engineering. Ann Biomed Eng, 32(3): 477–486.
24. Eivazzadeh‐Keihan R, Bahojb Noruzi E, Khanmohammadi Chenab K, et al., 2020, Metal‐based nanoparticles for bone tissue engineering. J Tissue Eng Regen Med, 14(12): 1687–1714.
25. Porter JR, Ruckh TT, Popat KC, 2009, Bone tissue engineering: A review in bone biomimetics and drug delivery strategies. Biotechnol Prog, 25(6): 1539–1560.
26. Kikuchi M, Itoh S, Ichinose S, et al., 2001, Self-organization mechanism in a bone-like hydroxyapatite/collagen nanocomposite synthesized in vitro and its biological reaction in vivo. Biomaterials, 22(13): 1705–1711.
27. Rezwan K, Chen Q, Blaker JJ, et al., 2006, Biodegradable and bioactive porous polymer/inorganic composite scaffolds for bone tissue engineering. Biomaterials, 27(18): 3413–3431.
28. Place ES, George JH, Williams CK, et al., 2009, Synthetic polymer scaffolds for tissue engineering. Chem Soc Rev, 38(4): 1139–1151.
29. Liu D, Nie W, Li D, et al., 2019, 3D printed PCL/SrHA scaffold for enhanced bone regeneration. Chem Eng J, 362: 269–279.
30. Cho YS, Quan M, Kang N-U, et al., 2020, Strategy for enhancing mechanical properties and bone regeneration of 3D polycaprolactone kagome scaffold: Nano hydroxyapatite composite and its exposure. Eur Polym J, 134: 109814.
31. Zhang Y, Yu W, Ba Z, et al., 2018, 3D-printed scaffolds of mesoporous bioglass/gliadin/polycaprolactone ternary composite for enhancement of compressive strength, degradability, cell responses and new bone tissue ingrowth. Int J Nanomed, 13: 5433.
32. Yang GH, Yeo M, Choi E, et al., 2021, Investigating the physical characteristics and cellular interplay on 3D-printed scaffolds depending on the incorporated silica size for hard tissue regeneration. Mater Des, 207: 109866.
33. Wu Y-HA, Chiu Y-C, Lin Y-H, et al., 2019, 3D-printedbioactive calcium silicate/poly-ε-caprolactone bioscaffolds modified with biomimetic extracellular matrices for bone regeneration. Int J Mol Sci, 20(4): 942.
34. Kim JA, Yun H-s, Choi Y-A, et al., 2018, Magnesium phosphate ceramics incorporating a novel indene compound promote osteoblast differentiation in vitro and bone regeneration in vivo. Biomaterials, 157: 51–61.
35. Jeon H, Yun S, Choi E, et al., 2019, Proliferation and osteogenic differentiation of human mesenchymal stem cells in PCL/silanated silica composite scaffolds for bone tissue regeneration. J Ind Eng Chem, 79: 41–51.
36. Zhou J, Chen K-M, Zhi D-J, et al., 2015, Effects of pyrite bioleaching solution of Acidithiobacillus ferrooxidans on viability, differentiation and mineralization potentials of rat osteoblasts. Arch Pharm Res, 38(12): 2228–2240.
37. Liu L, Zhao G, Gao Q, et al., 2017, Changes of mineralogical characteristics and osteoblast activities of raw and processed pyrites. RSC Adv, 7(45): 28373–28382.
38. Zhang L, Zheng Y, Xiong C, 2015, Fabrication and characterization of PDLLA/pyrite composite bone scaffold for osteoblast culture. Bull Mater Sci, 38(3): 811–816.
39. Pei Z, Zhang K, Li P, et al., 2020, Zi-Ran-Tong loaded brushite bone cement with enhanced osteoblast mineralization ability in vitro. Mater Lett, 259: 126908.
40. Feng W, Fu W-Y, Zhang Y, et al.,. 2004, Effects of Chinese herb medicine on the biological functions of cultured osteoblasts in vitro. Acad J Shanghai Second Med Univ, 24: 542–544.
41. Chakrapani VY, Gnanamani A, Giridev V, et al., 2012, Electrospinning of type I collagen and PCL nanofibers using acetic acid. J Appl Polym Sci, 125(4): 3221–3227.
42. Rath R, Subramanian S, Pradeep T, 2000, Surface chemical studies on pyrite in the presence of polysaccharide-based flotation depressants. J Colloid Interface Sci, 229(1): 82–91.
43. Lu J, Flautre B, Anselme K, et al., 1999, Role of interconnections in porous bioceramics on bone recolonization in vitro and in vivo. J Mater Sci Mater Med, 10(2): 111–120.
44. Bettinger CJ, Langer R, Borenstein JT, 2009, Engineering substrate topography at the micro‐and nanoscale to control cell function. Angew Chem Int Ed, 48(30): 5406–5415.
45. Loesberg W, Te Riet J, Van Delft F, et al., 2007, The threshold at which substrate nanogroove dimensions may influence fibroblast alignment and adhesion. Biomaterials, 28(27): 3944–3951.
46. Ivirico JE, Salmeron‐Sanchez M, Ribelles JG, et al., 2009, Proliferation and differentiation of goat bone marrow stromal cells in 3D scaffolds with tunable hydrophilicity. J Biomed Mater Res Part B Appl Biomater, 91(1): 277–286.
47. Jansen EJ, Sladek RE, Bahar H, et al., 2005, Hydrophobicity as a design criterion for polymer scaffolds in bone tissue engineering. Biomaterials, 26(21): 4423–4431.
48. Deng Y, Liu X, Xu A, et al., 2015, Effect of surface roughness on osteogenesis in vitro and osseointegration in vivo of carbon fiber-reinforced polyetheretherketone–nanohydroxyapatite composite. Int J Nanomed, 10: 1425.
49. Salgado AJ, Coutinho OP, Reis RL, 2004, Bone tissue engineering: state of the art and future trends. Macromol Biosci, 4(8): 743–765.
50. Spector M, 1994, Anorganic bovine bone and ceramic analogs of bone mineral as implants to facilitate bone regeneration. Clin Plast Surg, 21(3): 437–444.
51. Li C, Vepari C, Jin H-J, et al., 2006, Electrospun silk-BMP-2 scaffolds for bone tissue engineering. Biomaterials, 27(16): 3115–3124.
52. Riley EH, Lane JM, Urist MR, et al., 1996, Bone morphogenetic protein-2: Biology and applications. Clin Orthop Relat Res, 324: 39–46.
53. Kanczler J, Oreffo R, 2008, Osteogenesis and angiogenesis: The potential for engineering bone. Eur Cells Mater, 15(2): 100–114.
54. Zhu S, Bennett S, Kuek V, et al., 2020, Endothelial cells produce angiocrine factors to regulate bone and cartilage via versatile mechanisms. Theranostics, 10(13): 5957.
55. Köllmer M, Buhrman JS, Zhang Y, et al., 2013, Markers are shared between adipogenic and osteogenic differentiated mesenchymal stem cells. J Dev Biol Tissue Eng, 5(2): 18.
56. Franceschi R, 1999, The developmental control of osteoblast specific gene expression: Role of specific transcription factors and the extracellular matrix environment. Crit Rev Oral Biol Med, 10(1): 40–57.
57. Thurner PJ, Chen CG, Ionova-Martin S, et al., 2010, Osteopontin deficiency increases bone fragility but preserves bone mass. Bone, 46(6): 1564–1573.
58. Poundarik AA, Diab T, Sroga GE, et al., 2012, Dilatational band formation in bone. Proc Natl Acad Sci, 109(47): 19178–19183.
59. Wang Y, Wan C, Deng L, et al., 2007, The hypoxia-inducible factor α pathway couples angiogenesis to osteogenesis during skeletal development. J Clin Investig, 117(6): 1616–1626.