3D printing biocompatible materials with Multi Jet Fusion for bioreactor applications
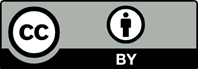
In the evolving three-dimensional (3D) printing technology, the involvement of different materials in any new 3D printing process necessitates a thorough evaluation of the product’s biocompatibility for biomedical application. Here, we examined the ability of Multi Jet Fusion (MJF)-printed PA-12 to support cell proliferation and osteogenesis. Our results show that leachate from MJF-printed PA-12 does not inhibit the growth of L929 fibroblast and MC3T3e1 osteoblast. The substrate supports the attachment and proliferation of both cell types, though not at a level comparable to conventional polystyrene culture plate. Neither plasma treatment, poly-D-lysine, nor collagen coatings narrowed the gap substantially, suggesting the possible influence of other limiting factors. The substrate can also support MC3T3e1 osteogenesis. However, MJF-printed PA-12 exhibits varying ability in supporting the proliferation of different cell types, especially in subsequent passages. While L929’s proliferation is comparable from passage-to-passage, MC3T3e1’s growth ability is noticeably compromised. Interestingly, our results show that L929 subcultured back to polystyrene plate retains the ability to grow as robustly as those on the conventional plate, suggesting that MJF-printed PA-12 does not permanently impair cell proliferation. In addition, we have shown the successful culture of bacterial Escherichia coli on MJF-printed PA-12. Together, our study demonstrated the potential of MJF-printed PA-12 for biological applications.
1. Shahrubudin N, Lee TC, Ramlan R, 2019, An overview on 3D printing technology: Technological, materials, and applications. Proc Manuf, 35: 1286–1296.
2. Buchanan C, Gardner L, 2019, Metal 3D printing in construction: A review of methods, research, applications, opportunities and challenges. Eng Struct, 180: 332–348. https://doi.org/10.1016/j.engstruct.2018.11.045
3. Chen Z, Li Z, Li J, et al., 2019, 3D printing of ceramics: A review. J Eur Ceram Soc, 39: 661–687.
4. Sikder P, Ferreira JA, Fakhrabadi EA, et al., 2020, Bhaduri, bioactive amorphous magnesium phosphate-polyetheretherketone composite filaments for 3D printing. Dent Mater, 36: 865–883.
5. Daminabo SC, Goel S, Grammatikos SA, et al., 2020, thakur, fused deposition modeling-based additive manufacturing (3D printing): Techniques for polymer material systems. Mater Today Chem, 16: 100248.
6. Singh B, Kumar R, Chohan JS, 2020, Polymer matrix composites in 3D printing: A state of art review. Mater Today, 33: 1562–1567.
7. Ligon SC, Liska R, Stampfl J, et al., 2017, Polymers for 3D printing and customized additive manufacturing. Chem Rev, 117: 10212–10290. https://doi.org/10.1021/acs.chemrev.7b00074
8. Stansbury JW, Idacavage MJ, 2016, 3D printing with polymers: Challenges among expanding options and opportunities. Dent Mater, 32: 54–64. https://doi.org/10.1016/j.dental.2015.09.018
9. Wang X, Jiang M, Zhou Z, et al., 2017, 3D printing of polymer matrix composites: A review and prospective. Compos Part B Eng, 110: 442–458. https://doi.org/10.1016/j.compositesb.2016.11.034
10. Ortiz-Acosta D, Moore T, 2018, Functional 3D printed polymeric materials. In: Functional materials. 9: 1-5. https://doi.org/10.5772/intechopen.80686
11. Nadgorny M, Ameli A, 2018, functional polymers and nanocomposites for 3D printing of smart structures and devices. ACS Appl Mater Interfaces, 10: 17489–17507.
12. Falahati M, Ahmadvand P, Safaee S, et al., 2020, Smart polymers and nanocomposites for 3D and 4D printing. Mater Today, 40: 215–245.
13. Bekas D, Hou Y, Liu Y, et al., 2019, 3D printing to enable multifunctionality in polymer-based composites: A review. Compos B Eng, 179:107540.
14. Sagias V, Giannakopoulos KI, Stergiou CI, 2018, Mechanical properties of 3D printed polymer specimens. Proc Struct Integr, 10: 85–90.
15. Ahangar P, Cooke ME, Weber MH, et al., 2019, Current biomedical applications of 3D printing and additive manufacturing. Appl Sci, 9: 1713.
16. Fan D, Li Y, Wang X, et al., 2020, Progressive 3D printing technology and its application in medical materials. Front Pharmacol, 11: 122. https://doi.org/10.3389/fphar.2020.00122
17. Ahmed S, Chauhan VM, Ghaemmaghami AM, et al., 2019, New generation of bioreactors that advance extracellular matrix modelling and tissue engineering. Biotechnol Lett, 41: 1–25. https://doi.org/10.1007/s10529-018-2611-7
18. Pantazis AK, Papadakis G, Parasyris K, et al., 2020, 3D-printed bioreactors for dna amplification: Application to companion diagnostics. Sens Actuators B Chem, 319: 128161. https://doi.org/10.1016/j.snb.2020.128161
19. Ferraz MA, Henning HH, Da Costa PF, et al., 2018, Potential health and environmental risks of three-dimensional engineered polymers. Environ Sci Technol Lett, 5: 80–85. https://doi.org/10.1021/acs.estlett.7b00495
20. Inoue Y, Ikuta K, 2013, Detoxification of the photocurable polymer by heat treatment for microstereolithography. Proc CIRP, 5: 115–118. https://doi.org/10.1016/j.procir.2013.01.023
21. Campanale C, Massarelli C, Savino I, et al., 2020, A detailed review study on potential effects of microplastics and additives of concern on human health. Int J Environ Res Public Health, 17: 1212. https://doi.org/10.3390/ijerph17041212
22. Ojeda T, 2013, Polymers and the environment. Polym Sci, 23: 23. https://doi.org/10.5772/51057
23. Priyadarshini BM, Dikshit V, Zhang Y, 2020, 3D-printed bioreactors for in vitro modeling and analysis. Int J Bioprint, 6: 267. https://doi.org/10.18063/ijb.v6i4.267
24. Zhang Y, 2017, post-printing surface modification and functionalization of 3D-printed biomedical device. Int J Bioprint, 3: 1. https://doi.org/10.18063/IJB.2017.02.001
25. Popov VK, Evseev AV, Ivanov A, et al., 2004, Laser stereolithography and supercritical fluid processing for custom-designed implant fabrication. J Mater Sci Mater Med, 15: 123–128. https://doi.org/10.1023/b: jmsm.0000011812.08185.2a
26. D’Urso PS, Effeney DJ, Earwaker WJ, et al., 2000, Custom cranioplasty using stereolithography and acrylic. Br J Plast Surg, 53: 200–204. https://doi.org/10.1054/bjps.1999.3268
27. Putame G, Gabetti S, Carbonaro D, et al., 2020, Compact and tunable stretch bioreactor advancing tissue engineering implementation. application to engineered cardiac constructs. Med Eng Phys, 84: 1–9. https://doi.org/10.1016/j.medengphy.2020.07.018
28. Rimington RP, Capel AJ, Chaplin KF, et al., 2019, Differentiation of bioengineered skeletal muscle within a 3D printed perfusion bioreactor reduces atrophic and inflammatory gene expression. ACS Biomater Sci Eng, 5: 5525–5538.
29. Banik BL, Brown JL, 2020, 3D-printed bioreactor enhances potential for tendon tissue engineering. Regen Eng Transl Med, 6: 1–10. https://doi.org/10.1007/s40883-019-00145-y
30. Smith LJ, Li P, Holland MR, et al., 2018, FABRICA: A bioreactor platform for printing, perfusing, observing, and stimulating 3D tissues. Sci Rep, 8: 1–10.
31. Achinas S, Euverink GJ, 2019, Development Of An Anaerobic Digestion Screening System Using 3D-Printed Mini-Bioreactors. In: New Adv Ferment Processes. London: Intechopen.
32. Xia C, Fang NX, 2009, 3D microfabricated bioreactor with capillaries. Biomed Microdev, 11: 1309–1315. https://doi.org/10.1007/s10544-009-9350-4
33. Zhou LY, Fu J, He Y, 2020, A review of 3D printing technologies for soft polymer materials. Adv Funct Mater, 30: 2000187. https://doi.org/10.1002/adfm.202000187
34. Tamay DG, Usal TD, Alagoz AS, et al., 2019, 3D and 4D printing of polymers for tissue engineering applications. Front Bioeng Biotechnol, 7: 164–164. https://doi.org/10.3389/fbioe.2019.00164
35. Tagliaferri V, Trovalusci F, Guarino S, et al., 2019, Environmental and economic analysis of fdm, sls and mjf additive manufacturing technologies. Materials (Basel), 12: 4161. https://doi.org/10.3390/ma12244161
36. Calignano F, Galati M, Iuliano L, et al., 2019, design of additively manufactured structures for biomedical applications: A review of the additive manufacturing processes applied to the biomedical sector. J Healthc Eng, 2019: 9748212. https://doi.org/10.1155/2019/9748212
37. Rahim TN, Abdullah AM, Akil HM, et al., 2016, Comparison of mechanical properties for polyamide 12 composite-based biomaterials fabricated by fused filament fabrication and injection molding. AIP Conf Proc, 1791: 020007 https://doi.org/10.1063/1.4968862
38. Feng L, Wang Y, Wei Q, 2019, PA12 powder recycled from SLS for FDM. Polymers, 11: 727. https://doi.org/10.3390/polym11040727
39. Kinstlinger IS, Bastian A, Paulsen SJ, et al., 2016, Open-source selective laser sintering (OpenSLS) of nylon and biocompatible polycaprolactone. PLoS One, 11: e0147399. https://doi.org/10.1371/journal.pone.0147399
40. Shi Y, Pan T, Zhu W, et al., 2020, Artificial bone scaffolds of coral imitation prepared by selective laser sintering. J Mech Behav Biomed Mater, 104: 103664. https://doi.org/10.1016/j.jmbbm.2020.103664
41. Gan X, Wang J, Wang Z, et al., 2019, Simultaneous realization of conductive segregation network microstructure and minimal surface porous macrostructure by SLS 3D printing. Mater Des, 178: 107874.
42. Hussain G, Khan WA, Ashraf HA, et al., 2019, Design and development of a lightweight SLS 3D printer with a controlled heating mechanism: Part A. Int J Lightweight Mater Manuf, 2: 373–378. https://doi.org/10.1016/j.ijlmm.2019.01.005
43. Cai C, Tey WS, Chen J, et al., 2021, Comparative study on 3D printing of polyamide 12 by selective laser sintering and multi jet fusion. J Mater Process Technol, 288: 116882. https://doi.org/10.1016/j.jmatprotec.2020.116882
44. Chin SY, Dikshit V, Priyadarshini BM, et al., 2020, Powder-based 3D printing for the fabrication of device with micro and mesoscale features. Micromachines (Basel), 11: 658. https://doi.org/10.3390/mi11070658
45. Dikshit V, Goh GD, Nagalingam AP, et al., 2020, Recent progress in 3D printing of fiber-reinforced composite and nanocomposites. In: Han B, Sharma S, Nguyen TA, editors. Fiber-Reinforced Nanocomposites: Fundamentals and Applications. Ch. 17. Amsterdam: Elsevier, p371-p394.
46. Yuan S, Shen F, Chua CK, et al., 2019, Polymeric composites for powder-based additive manufacturing: Materials and applications. Prog Polym Sci, 91: 141–168.https://doi.org/10.1016/j.progpolymsci.2018.11.001
47. Wu H, Fahy W, Kim S, et al., 2020, Recent developments in polymers/polymer nanocomposites for additive manufacturing. Prog Mater Sci, 111: 100638. https://doi.org/10.1016/j.pmatsci.2020.100638
48. Wang H, Li Y, Zuo Y, et al., 2007, Biocompatibility and osteogenesis of biomimetic Nano-hydroxyapatite/ polyamide composite scaffolds for bone tissue engineering. Biomaterials, 28: 3338–3348. https://doi.org/10.1016/j.biomaterials.2007.04.014
49. Upadhyay DJ, Cui NY, Anderson CA, et al., 2004, A comparative study of the surface activation of polyamides using an air dielectric barrier discharge. Colloids Surf Physicochem Eng Aspects, 248: 47–56.
https://doi.org/10.1016/j.colsurfa.2004.08.016
50. Goodridge RD, Tuck CJ, Hague RJ, 2012, Laser sintering of polyamides and other polymers. Prog Mater Sci, 57: 229–267. https://doi.org/10.1016/j.pmatsci.2011.04.001
51. Liu G, Li Y, Yang L, et al., 2017, Cytotoxicity study of polyethylene glycol derivatives. RSC Adv, 7: 18252–18259.
52. Yuan X, Zhang X, Sun L, et al., 2019, Cellular toxicity and immunological effects of Carbon-based nanomaterials. Part Fibre Toxicol, 16: 18.
53. Rosso S, Meneghello R, Biasetto L, et al., 2020, In-depth comparison of polyamide 12 parts manufactured by multi jet fusion and selective laser sintering. Addit Manuf, 36: 101713. https://doi.org/10.1016/j.addma.2020.101713
54. Scherer B, Kottenstedde IL, Matysik FM, 2020, Material characterization of polyamide 12 and related agents used in the multi-jet fusion process: Complementary application of high-resolution mass spectrometry and other advanced instrumental techniques. Monatsh Chem Chem Mon, 151: 1203–1215.
55. Boyde A, Wood C, 1969, Preparation of animal tissues for Surface-scanning electron microscopy. J Microsc, 90: 221–249. https://doi.org/10.1111/j.1365-2818.1969.tb00709.x
56. Ulmer K, Honjo S, 1973, Quantitative evaluation of fixation and dehydration methods for scanning electron microscopic preparation of soft sea water organisms. In: Proceeding 6th annual SEM symposium, p365.
57. Bessis M, Weed R, 1972, Preparation of red blood cells (RBC) for SEM. A survey of various artifacts. Scan Electron Microsc, 1: 290–296.
58. Waterman RE, 1972, Use of the scanning electron microscope for observation of vertebrate embryos. Dev Biol, 27: 276–81. https://doi.org/10.1016/0012-1606(72)90103-0
59. Lin SD, Lamvik M, 1975, High resolution scanning electron microscopy at the subcellular level. J Microsc, 103: 249–257. https://doi.org/10.1111/j.1365-2818.1975.tb03900.x
60. O’Connor HJ, Dickson AN, Dowling DP, 2018, Evaluation of the mechanical performance of polymer parts fabricated using a production scale multi jet fusion printing process. Addit Manuf, 22: 381–387. https://doi.org/10.1016/j.addma.2018.05.035
61. Palma T, Munther M, Damasus P, et al., 2019, Multiscale mechanical and tribological characterizations of additively manufactured polyamide 12 parts with different print orientations. J Manuf Processes, 40: 76–83. https://doi.org/10.1016/j.jmapro.2019.03.004
62. Dadbakhsh S, Verbelen L, Verkinderen O, et al., 2017, Effect of PA12 powder reuse on coalescence behaviour and microstructure of SLS parts. Eur Polym J, 92: 250–262. https://doi.org/10.1016/j.eurpolymj.2017.05.014
63. Yusoff W, Thomas A, 2008, The effect of employing an effective laser sintering scanning strategy and energy density value on eliminating “Orange peel” on a selective laser sintered part. International association for management of technology, proceedings.
64. Holländer A, Cosemans P, 2020, Surface technology for additive manufacturing. Plasma Processes Polym, 17: 1900155. https://doi.org/10.1002/ppap.201900155
65. Cai S, Wu C, Yang W, et al., 2020, Recent advance in surface modification for regulating cell adhesion and behaviors. Nanotechnol Rev, 9: 971–989. https://doi.org/10.1515/ntrev-2020-0076
66. Wieslander AP, Nordin MK, Hansson B, et al., 1993, In vitro toxicity of biomaterials determined with cell density, total protein, cell cycle distribution and adenine nucleotides. Biomater Artif Cells Immobilization Biotechnol, 21: 63–70. https://doi.org/10.3109/10731199309118297
67. Kumar P, Nagarajan A, Uchil PD, 2018, Analysis of cell viability by the MTT assay. Cold Spring Harb Protoc, 2018: pdb.prot095505.
68. Alimohamadi M, Ownagh V, Mahouzi L, et al., 2014, The impact of immunohistochemical markers of ki-67 and p53 on the Long-term outcome of growth Hormone-secreting pituitary adenomas: A cohort study. Asian J Neurosurg, 9:130–136. https://doi.org/10.4103/1793-5482.142732
69. El Badawi ZH, Muhammad EM, Noaman HH, 2015, Utility of Ki-67 labeling index in the differential diagnosis of osteogenic bone tumors. Al-Azhar Assiut Med J, 13: 1687–1693.
70. Yan XZ, Yang W, Yang F, et al., 2014, Effects of continuous passaging on mineralization of MC3T3-E1 cells with improved osteogenic culture protocol. Tissue Eng Part C Methods, 20: 198–204. https://doi.org/10.1089/ten.tec.2012.0412
71. Liao H, He H, Chen Y, et al., 2014, Effects of Long-term serial cell passaging on cell spreading, migration, and Cell-surface ultrastructures of cultured vascular endothelial cells. Cytotechnology, 66: 229–238. https://doi.org/10.1007/s10616-013-9560-8
72. Hughes FJ. 1998, Aubin JE, 1998, Culture of cells of the osteoblast lineage. Methods Bone Biol, 22: 1–49.
73. Thio BJ, Meredith JC, 2008, Quantification of E. coli adhesion to polyamides and polystyrene with atomic force microscopy. Colloids Surf B Biointerfaces, 65: 308–312. https://doi.org/10.1016/j.colsurfb.2008.05.005
74. Wang H, Feng H, Liang W, et al., 2009, Malyarchuk, effect of surface roughness on retention and removal of Escherichia coli O157: H7 on surfaces of selected fruits. J Food Sci, 74: E8–E15. https://doi.org/10.1111/j.1750-3841.2008.00998.x