3D-Printed β-Tricalcium Phosphate Scaffolds Promote Osteogenic Differentiation of Bone Marrow-Deprived Mesenchymal Stem Cells in an N6-methyladenosineDependent Manner
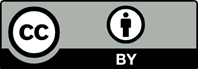
Bone defect is a serious orthopedic disease which has been studied for a long time. Alternative degradable biomaterials are required for bone repairing and regeneration to address the limitation of autogenous bone. β-tricalcium phosphate (β-TCP) is an alternative material with good cytocompatibility and has been used in bone defect treatment. However, whether β-TCP contributes to osteogenesis of bone marrow stem cells (BMSCs) through N6-methyladenosine (m6A) modification remains unknown. To address this issue, we verified the effects of β-TCP on osteogenesis of BMSCs. We also studied the expression of m6A-related enzymes in BMSCs after β-TCP treatment. Furthermore, the m6A level and stability of Runt-related transcription factor 2 (RUNX2) mRNA were investigated after β-TCP treatment. Finally, rat calvarial defect models were performed to detect expression level of osteogenic factors and m6A-related enzymes after the stimulation of three-dimension (3D)-printed β-TCP scaffolds. We found that β-TCP showed good biocompatibility and was osteoinductive. Meanwhile, methyltransferase-like 3 (METTL3) increased, causing the elevation of m6A level of RUNX2, results in stabler RUNX2 mRNA level. At last, based on the animal experiments, we demonstrated that the increase of RUNX2 and METTL3 levels was induced by β-TCP. These findings suggest that METTL3 increases the m6A level of RUNX2 mRNA after β-TCP induction, contributing to its stability, and the results in vivo also confirmed the osteogenic and bone-repair properties of β-TCP.
1. Wang Z, Guo Z, Bai H, et al., 2013, Clinical Evaluation of β-TCP in the Treatment of Lacunar Bone Defects: A Prospective, Randomized Controlled Study. Mater Sci Eng C, 33:1894–9. https://doi.org/10.1016/j.msec.2012.12.041
2. Seebach C, Henrich D, Kähling C, et al., 2010, Endothelial Progenitor Cells and Mesenchymal Stem Cells Seeded onto β-TCP Granules Enhance Early Vascularization and Bone Healing in a Critical-Sized Bone Defect in Rats. Tissue Eng Part A, 16:1961–70. https://doi.org/10.1089/ten.tea.2009.0715
3. Chu W, Zhuang Y, Gan Y, et al., 2019, Comparison and Characterization of Enriched Mesenchymal Stem Cells Obtained by the Repeated Filtration of Autologous Bone Marrow Through Porous Biomaterials. J Transl Med, 17:377. https://doi.org/10.1186/s12967-019-02131-y
4. Chu W, Gan Y, Zhuang Y, et al., 2018, Mesenchymal Stem Cells and Porous β-tricalcium Phosphate Composites Prepared through Stem Cell Screen-enrich-combine (Biomaterials) Circulating System for the Repair of Critical Size Bone Defects in Goat Tibia. Stem Cell Res Ther, 9:157. https://doi.org/10.1186/s13287-018-0906-1
5. Chu W, Wang X, Gan Y, et al., 2019, Screen-enrich-combine Circulating System to Prepare MSC/β-TCP for Bone Repair in Fractures with Depressed Tibial Plateau. Regener Med, 14:555–69. https://doi.org/10.2217/rme-2018-0047
6. Wang X, Chu W, Zhuang Y, et al., 2019, Bone Mesenchymal Stem Cell-Enriched β-Tricalcium Phosphate Scaffold Processed by the Screen-Enrich-Combine Circulating System Promotes Regeneration of Diaphyseal Bone Non-Union. Cell Transplant, 28:212–23. https://doi.org/10.1177/0963689718818096
7. Masaoka T, Yoshii T, Yuasa M, et al., 2016, Bone Defect Regeneration by a Combination of a β-Tricalcium Phosphate Scaffold and Bone Marrow Stromal Cells in a Non-Human Primate Model. Open Biomed Eng J, 10: 2-11. https://doi.org/10.2174/1874120701610010002
8. Barradas AM, Monticone V, Hulsman M, et al., 2013, Molecular Mechanisms of Biomaterial-driven Osteogenic Differentiation in Human Mesenchymal Stromal Cells. Integr Biol, 5:920–31. https://doi.org/10.1039/c3ib40027a
9. Liu J, Zhao L, Ni L, et al., 2015, The Effect of Synthetic α-tricalcium Phosphate on Osteogenic Differentiation of Rat Bone Mesenchymal Stem Cells. Am J Transl Res, 7:1588–601.
10. Rittipakorn P, Thuaksuban N, Mai-Ngam K, et al., 2021, Bioactivity of a Novel Polycaprolactone-Hydroxyapatite Scaffold Used as a Carrier of Low Dose BMP-2: An In Vitro Study. Polymers (Basel), 13:466. https://doi.org/10.3390/polym13030466
11. Hesse E, Saito H, Kiviranta R, et al., 2010, Zfp521 Controls Bone Mass by HDAC3-dependent Attenuation of Runx2 Activity. J Cell Biol, 191:1271–83. https://doi.org/10.1083/jcb.201009107
12. Liu JC, Lengner CJ, Gaur T, et al., 2011, Runx2 Protein Expression Utilizes the Runx2 P1 Promoter to Establish Osteoprogenitor Cell Number for Normal Bone Formation. J Biol Chem, 286:30057–70. https://doi.org/10.1074/jbc.M111.241505
13. Kronenberg HM, 2004, Twist Genes Regulate Runx2 and Bone Formation. Dev Cell, 6:317–8. https://doi.org/10.1016/S1534-5807(04)00069-3
14. Yan G, Yuan Y, He M, et al., 2020, m6A Methylation of Precursor-miR-320/RUNX2 Controls Osteogenic Potential of Bone Marrow-Derived Mesenchymal Stem Cells. Mol Ther Nucleic Acids, 19:421–36. https://doi.org/10.1016/j.omtn.2019.12.001
15. Chen J, Tian Y, Zhang Q, et al., 2021, Novel Insights into the Role of N6-Methyladenosine RNA Modification in Bone Pathophysiology. Stem Cells Dev, 30:17–28. https://doi.org/10.1089/scd.2020.0157
16. Lee M, Kim B, Kim VN, 2014, Emerging Roles of RNA Modification: m 6 A and U-Tail. Cell, 158:980–7. https://doi.org/10.1016/j.cell.2014.08.005
17. Zhao BS, Roundtree IA, He C, 2017, Post-transcriptional Gene Regulation by mRNA Modifications. Nat Rev Mol Cell Biol, 18:31–42. https://doi.org/10.1038/nrm.2016.132
18. He C, 2010, Grand Challenge Commentary: RNA epigenetics? Nat Chem Biol, 6:863–65. https://doi.org/10.1038/nchembio.482
19. Yang Y, Hsu PJ, Chen YS, et al., 2018, Dynamic Transcriptomic m6A Decoration: Writers, Erasers, Readers and Functions in RNA Metabolism. Cell Res, 28:616–24. https://doi.org/10.1038/s41422-018-0040-8
20. Wu Y, Xie L, Wang M, et al., 2018, Mettl3-mediated m6A RNA Methylation regulates the fate of bone marrow mesenchymal stem cells and osteoporosis. Nat Commun, 9:4772. https://doi.org/10.1038/s41467-018-06898-4
21. Yu J, Shen L, Liu Y, et al., 2020, The m6A Methyltransferase METTL3 Cooperates with Demethylase ALKBH5 to Regulate Osteogenic Differentiation through NF-Κb Signaling. Mol Cell Biochem, 463:203–10. https://doi.org/10.1007/s11010-019-03641-5
22. Liu Y, Gu C, Li X, et al., 2021, Involvement of METTL3/m6Adenosine and TGFβ/Smad3 Signaling on Tenon’s Fibroblasts and in a Rabbit Model of Glaucoma Surgery. J Mol Histol, 52:1129–44. https://doi.org/10.1007/s10735-021-10028-8
23. Liu L, Yu F, Li L, et al., 2021, Bone Marrow Stromal Cells Stimulated by Strontium-substituted Calcium Silicate Ceramics: Release of Exosomal miR-146a Regulates Osteogenesis and Angiogenesis. Acta Biomater, 119:444–57. https://doi.org/10.1016/j.actbio.2020.10.038
24. Zhou P, Xia D, Ni Z, et al., 2021, Calcium Silicate Bioactive Ceramics Induce Osteogenesis through Oncostatin M. Bioact Mater, 6:810–22. https://doi.org/10.1016/j.bioactmat.2020.09.018
25. Huang Y, Jin X, Zhang X, et al., 2009, In Vitro and In Vivo Evaluation of Akermanite Bioceramics for Bone Regeneration. Biomaterials, 30:5041–8. https://doi.org/10.1016/j.biomaterials.2009.05.077
26. Sun X, Ma Z, Zhao X, et al., 2021, Three-dimensional Bioprinting of Multicell-laden Scaffolds Containing Bone Morphogenic Protein-4 for Promoting M2 Macrophage Polarization and Accelerating Bone Defect Repair in Diabetes Mellitus. Bioact Mater, 6:757–69. https://doi.org/10.1016/j.bioactmat.2020.08.030
27. Zheng M, Weng M, Zhang X, et al., 2021, Beta-tricalcium Phosphate Promotes Osteogenic Differentiation of Bone Marrow-derived Mesenchymal Stem Cells through Macrophages. Biomed Mater, 16:025005. https://doi.org/10.1088/1748-605X/abdbdc
28. El-Rashidy AA, Roether JA, Harhaus L, et al., 2017, Regenerating Bone with Bioactive Glass Scaffolds: A Review of In Vivo Studies in Bone Defect Models. Acta Biomater, 62:1–28. https://doi.org/10.1016/j.actbio.2017.08.030
29. Wu D, Wang Z, Wang J, et al., 2018, Development of a Micro-tissue-mediated Injectable Bone Tissue Engineering Strategy for Large Segmental Bone Defect Treatment. Stem Cell Res Ther, 9:331. https://doi.org/10.1186/s13287-018-1064-1
30. Stevens MM, 2008, Biomaterials for Bone Tissue Engineering. Mater Today, 11:18–25. https://doi.org/10.1016/S1369-7021(08)70086-5
31. Baldwin P, Li DJ, Auston DA, et al., 2019, Autograft, Allograft, and Bone Graft Substitutes: Clinical Evidence and Indications for Use in the Setting of Orthopaedic Trauma Surgery. J Orthop Trauma, 33:203–13. https://doi.org/10.1097/BOT.0000000000001420
32. Prakash J, Prema D, Venkataprasanna KS, et al., 2020, Nanocomposite Chitosan Film Containing Graphene Oxide/Hydroxyapatite/Gold for Bone Tissue Engineering. Int J Biol Macromol, 154:62–71. https://doi.org/10.1016/j.ijbiomac.2020.03.095
33. Kaushik N, Nguyen LN, Kim JH, et al., 2020, Strategies for Using Polydopamine to Induce Biomineralization of Hydroxyapatite on Implant Materials for Bone Tissue Engineering. Int J Mol Sci, 21:E6544. https://doi.org/10.3390/ijms21186544
34. Take Y, Mae T, Yoneda M, et al., 2020, On-lay Grafting of a Calcium Hydroxyapatite Bone Substitute: A Preliminary Animal Experimental Study. J Orthop Sci, 25:1101–6. https://doi.org/10.1016/j.jos.2019.12.012
35. Sathiyavimal S, Vasantharaj S, Oscar FL, et al., 2019, Biosynthesis and Characterization of Hydroxyapatite and its Composite (Hydroxyapatite-gelatin-chitosan-fibrin-bone Ash) for Bone Tissue Engineering Applications. Int J Biol Macromol, 129:844–52. https://doi.org/10.1016/j.ijbiomac.2019.02.058
36. Beuriat PA, Lohkamp LN, Szathmari A, et al., 2019, Repair of Cranial Bone Defects in Children Using Synthetic Hydroxyapatite Cranioplasty (CustomBone). World Neurosurg, 129:e104–13. https://doi.org/10.1016/j.wneu.2019.05.052
37. Zhang H, Zhou Y, Yu N, et al., 2019, Construction of Vascularized Tissue-engineered Bone with Polylysine modified Coral Hydroxyapatite and a Double Cell-sheet Complex to Repair a Large Radius Bone Defect in Rabbits. Acta Biomater, 91:82–98. https://doi.org/10.1016/j.actbio.2019.04.024
38. Przekora A, Kazimierczak P, Wojcik M, 2021, Ex Vivo Determination of Chitosan/Curdlan/Hydroxyapatite Biomaterial Osseointegration with the Use of Human Trabecular Bone Explant: New Method for Biocompatibility Testing of Bone Implants Reducing Animal Tests. Mater Sci Eng C Mater Biol Appl, 119:111612. https://doi.org/10.1016/j.msec.2020.111612
39. Putri TS, Hayashi K, Ishikawa K, 2020, Bone Regeneration Using β-tricalcium Phosphate (β-TCP) block with Interconnected Pores Made by Setting Reaction of β-TCP Granules. J Biomed Mater Res A, 108:625–32. https://doi.org/10.1002/jbm.a.36842
40. Zhang D, Gao P, Li Q, et al., Engineering Biomimetic Periosteum with β-TCP Scaffolds to Promote Bone Formation in Calvarial Defects of Rats. Stem Cell Res Ther, 8:134. https://doi.org/10.1186/s13287-017-0592-4
41. Tian C, Huang Y, Li Q, et al., 2019, Mettl3 Regulates Osteogenic Differentiation and Alternative Splicing of Vegfa in Bone Marrow Mesenchymal Stem Cells. Int J Mol Sci, 20:551. https://doi.org/10.3390/ijms20030551
42. Son HE, Min HY, Kim EJ, et al., 2020, Fat Mass and Obesity-Associated (FTO) Stimulates Osteogenic Differentiation of C3H10T1/2 Cells by Inducing Mild Endoplasmic Reticulum Stress via a Positive Feedback Loop with p-AMPK. Mol Cells, 43:58–65.
43. Zhang C, Chen Y, Sun B, et al., 2017, m6A Modulates Haematopoietic Stem and Progenitor Cell Specification. Nature, 549:273–6. https://doi.org/10.1038/nature23883
44. Zhang B, Wu Q, Li B, et al., 2020, m6A Regulator-Mediated Methylation Modification Patterns and Tumor Microenvironment Infiltration Characterization in Gastric Cancer. Mol Cancer, 19:53. https://doi.org/10.1186/s12943-020-01170-0
45. Zhang Y, Gu X, Li D, et al., 2019, METTL3 Regulates Osteoblast Differentiation and Inflammatory Response via Smad Signaling and MAPK Signaling. Int J Mol Sci, 21:199. https://doi.org/10.3390/ijms21010199
46. Barbieri I, Tzelepis K, Pandolfini L, et al., 2017, Promoter bound METTL3 Maintains Myeloid Leukaemia by m6Adependent Translation Control. Nature, 552:126–31. https://doi.org/10.1038/nature24678
47. Dominissini D, Moshitch-Moshkovitz S, Schwartz S, et al., 2012, Topology of the Human and Mouse m6A RNA Methylomes Revealed by m6A-seq. Nature, 485:201–6. https://doi.org/10.1038/nature11112
48. Bartosovic M, Molares HC, Gregorova P, et al., 2017, N6-methyladenosine Demethylase FTO Targets pre-mRNAs and Regulates Alternative Splicing and 3’-end Processing. Nucleic Acids Res, 45:11356–70. https://doi.org/10.1093/nar/gkx778
49. Geula S, Moshitch-Moshkovitz S, Dominissini D, et al., 2015, Stem Cells. m6A mRNA Methylation Facilitates Resolution of Naïve Pluripotency toward Differentiation. Science, 347:1002–6. https://doi.org/10.1126/science.1261417
50. Kennedy EM, Bogerd HP, Kornepati AV, et al., 2017, Posttranscriptional m6A Editing of HIV-1 mRNAs Enhances Viral Gene Expression. Cell Host Microbe, 22:830. https://doi.org/10.1016/j.chom.2017.11.010