A Review on Design and Mechanical Properties of Additively Manufactured NiTi Implants for Orthopedic Applications
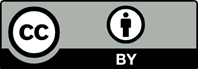
NiTi alloy has a wide range of applications as a biomaterial due to its high ductility, low corrosion rate, and favorable biocompatibility. Although Young’s modulus of NiTi is relatively low, it still needs to be reduced; one of the promising ways is by introducing porous structure. Traditional manufacturing processes, such as casting, can hardly produce complex porous structures. Additive manufacturing (AM) is one of the most advanced manufacturing technologies that can solve impurity issues, and selective laser melting (SLM) is one of the well-known methods. This paper reviews the developments of AM-NiTi with a particular focus on SLM-NiTi utilization in biomedical applications. Correspondingly, this paper aims to describe the three key factors, including powder preparation, processing parameters, and gas atmosphere during the overall process of porous NiTi. The porous structure design is of vital importance, so the unit cell and pore parameters are discussed. The mechanical properties of SLM-NiTi, such as hardness, compressive strength, tensile strength, fatigue behavior, and damping properties and their relationship with design parameters are summarized. In the end, it points out the current challenges. Considering the increasing application of NiTi implants, this review paper may open new frontiers for advanced and modern designs.
1. Lu B, Cui X, Jin G, et al., 2020, Effect of La2O3 Addition on Mechanical Properties and Wear Behaviour of NiTi Alloy Fabricated by Direct Metal Deposition. Opt Laser Technol, 129:106290. https://doi.org/10.1016/j.optlastec.2020.106290.
2. Mahtabi MJ, Shamsaei N, Mitchell MR, 2015, Fatigue of Nitinol: The State-of-the-Art and Ongoing Challenges. J Mech Behav Biomed Mater, 50:228–54. https://doi.org/10.1016/j.jmbbm.2015.06.010.
3. Wang L, Lu W, Qin J, et al., 2009, Effect of Precipitation Phase on Microstructure and Superelasticity of Cold-Rolled Beta Titanium Alloy During Heat Treatment. Mater Des, 30:3873–78. https://doi.org/10.1016/j.matdes.2009.03.042.
4. Wang L, Lu W, Qin J, et al., 2010, The Characterization of Shape Memory Effect for Low Elastic Modulus Biomedical β-Type Titanium Alloy. Mater Charact, 61:535–41. https://doi.org/10.1016/j.matchar.2010.02.009.
5. Bhagyaraj J, Ramaiah KV, Saikrishna CN, et al., 2013, Behavior and Effect of Ti2Ni Phase During Processing of NiTi Shape Memory Alloy Wire from Cast Ingot. J Alloys Compd, 581:344–51. https://doi.org/10.1016/j.jallcom.2013.07.046.
6. van Humbeeck J, 1999, Non-medical Applications of Shape Memory Alloys. Mater Sci Eng A, 275:134–48.
7. Sharma N, Raj T, Kumar K, 2015, Applications of Nickel-Titanium Alloy. J Eng Technol, 5:1–7.
8. Kumar PK, Lagoudas DC, 2008, Shape Memory Alloys, Modelling and Engineering Applications. Springer, TX, USA, pp. 1–51.
9. Wang X, Speirs M, Kustov S, et al., 2018, Selective Laser Melting Produced Layer-Structured NiTi Shape Memory Alloys with High Damping Properties and Elinvar Effect. Scr Mater, 146:246–50. https://doi.org/10.1016/j.scriptamat.2017.11.047.
10. Shariat BS, Meng Q, Mahmud AS, et al., 2017, Functionally Graded Shape Memory Alloys: Design, Fabrication and Experimental Evaluation. Mater Des, 124:225–37.
11. Wang J, Pan Z, Carpenter K, et al., 2021, Comparative Study on Crystallographic Orientation, Precipitation, Phase Transformation and Mechanical Response of Ni-Rich NiTi Alloy Fabricated by WAAM at Elevated Substrate Heating Temperatures. Mater Sci Eng A Struct, 800:140307. https://doi.org/10.1016/j.msea.2020.140307.
12. Wang L, Wang C, Lu W, et al., 2015, Superelasticity of NiTi-Nb Metallurgical Bonding Via Nanoindentation Observation. Mater Lett, 161:255–8. https://doi.org/10.1016/j.matlet.2015.08.089.
13. Wang L, Wang C, Zhang LC, et al., 2016, Phase Transformation and Deformation Behavior of NiTi-Nb Eutectic Joined NiTi Wires. Sci Rep, 6:23905. https://doi.org/10.1038/srep23905.
14. Liu S, Liu J, Wang L, et al., 2020, Superelastic Behavior of In Situ Eutectic-Reaction Manufactured High Strength 3D Porous NiTi-Nb Scaffold. Scr Mater, 181:121–6. https://doi.org/10.1016/j.scriptamat.2020.02.025.
15. Liu S, Han S, Zhang L, et al., 2020, Strengthening Mechanism and Micropillar Analysis of High-Strength NiTi-Nb Eutectic-Type Alloy Prepared by Laser Powder Bed Fusion. Compos B Eng, 200:108358. https://doi.org/10.1016/j.compositesb.2020.108358.
16. Wang L, Xie L, Zhang LC, et al., 2018, Microstructure Evolution and Superelasticity of Layer-Like NiTiNb Porous Metal Prepared by Eutectic Reaction. Acta Mater, 143:214–26. https://doi.org/10.1016/j.actamat.2017.10.021.
17. Bansiddhi A, Sargeant TD, Stupp SI, et al., 2008, Porous NiTi for Bone Implants: A Review. Acta Biomater, 4:773–82. https://doi.org/10.1016/j.actbio.2008.02.009.
18. Geetha M, Singh AK, Asokamani R, et al., 2009, Ti Based Biomaterials, the Ultimate Choice for Orthopaedic Implants-a Review. Prog Mater Sci, 54:397–425. https://doi.org/10.1016/j.pmatsci.2008.06.004.
19. Fischer M, Joguet D, Robin G, et al., 2016, In Situ Elaboration of a Binary Ti-26Nb Alloy by Selective Laser Melting of Elemental Titanium and Niobium Mixed Powders. Mater Sci Eng C Mater Biol Appl, 62:852–9. https://doi.org/10.1016/j.msec.2016.02.033.
20. Torres Y, Trueba P, Pavón JJ, et al., 2016, Design, Processing and Characterization of Titanium with Radial Graded Porosity for Bone Implants. Mater Des, 110:179–87. https://doi.org/10.1016/j.matdes.2016.07.135.
21. Simske SJ, Ayers RA, Bateman TA, 1997, Porous Materials for Bone Engineering. Porous Mater Tissue Eng, 250:151–82. https://doi.org/10.4028/www.scientific.net/msf.250.151.
22. Wang P, Li X, Jiang Y, et al., 2020, Electron Beam Melted Heterogeneously Porous Microlattices for Metallic Bone Applications: Design and Investigations of Boundary and Edge Effects. Addit Manuf, 36:101566. https://doi.org/10.1016/j.addma.2020.101566.
23. Li F, Li J, Xu G, et al., 2015, Fabrication, Pore Structure and Compressive Behavior of Anisotropic Porous Titanium for Human Trabecular Bone Implant Applications. J Mech Behav Biomed Mater, 46:104–14. https://doi.org/10.1016/j.jmbbm.2015.02.023.
24. Attarilar S, Ebrahimi M, Djavanroodi F, et al., 2021, 3D Printing Technologies in Metallic Implants: A Thematic Review on the Techniques and Procedures. Int J Bioprint, 7:306. https://doi.org/10.18063/ijb.v7i1.306.
25. Elahinia MH, Hashemi M, Tabesh M, et al., 2012, Manufacturing and Processing of NiTi Implants: A Review. Prog Mater Sci, 57:911–46. https://doi.org/10.1016/j.pmatsci.2011.11.001.
26. Wu MH, 2001, Fabrication of Nitinol Materials and Components. Mater Sci Forum, 394–395:285–92.
27. Aydoǧmus T, Bor AS, 2011, Production and Characterization of Porous TiNi Shape Memory Alloys. Turk J Eng Environ Sci, 35:69–82.
28. Ma C, Gu D, Dai D, et al., 2018, Selective Growth of Ni4Ti3 Precipitate Variants Induced by Complicated Cyclic Stress During Laser Additive Manufacturing of NiTi-Based Composites. Mater Charact, 143:191–6. https://doi.org/10.1016/j.matchar.2018.04.004.
29. Parvizi S, Hashemi SM, Asgarinia F, et al., 2020, Effective Parameters on the Final Properties of NiTi-based Alloys Manufactured by Powder Metallurgy Methods: A Review. Prog Mater Sci, 117:100739. https://doi.org/10.1016/j.pmatsci.2020.100739.
30. Yusuf SM, Gao N, 2017, Influence of Energy Density on Metallurgy and Properties in Metal Additive Manufacturing. Mater Sci Tech Lond, 33:1269–89.
31. Otubo J, Rigo OD, Moura NC, et al., 2003, Scale Up of NiTi Shape Memory Alloy Production by EBM. J Phys, 112:873–6. https://doi.org/10.1051/jp4:20031020.
32. Harun WS, Kamariah MS, Muhamad N, et al., 2018, A Review of Powder Additive Manufacturing Processes for Metallic Biomaterials. Powder Technol, 327:128–51. https://doi.org/10.1016/j.powtec.2017.12.058.
33. Wang P, Goh MH, Li Q, et al., 2020, Effect of Defects and Specimen Size with Rectangular Cross-Section on the Tensile Properties of Additively Manufactured Components. Virtual Phys Prototyp, 15:251–64. https://doi.org/10.1080/17452759.2020.1733430.
34. Sadrnezhaad SK, Ahmadi E, Malekzadeh M, 2009, Mechanism of Reaction of Molten NiTi with EBM Graphite Crucible. Mater Sci Technol, 25:699–706. https://doi.org/10.1179/174328408x317075.
35. Hayat MD, Chen G, Khan S, et al., 2018, Physical and Tensile Properties of the NiTi Alloy by Selective Electron Beam Melting. Key Eng Mater, 770:148–54. https://doi.org/10.4028/www.scientific.net/kem.770.148.
36. Dutkiewicz J, Rogal Ł, Kalita D, et al., 2020, Superelastic Effect in NiTi Alloys Manufactured Using Electron Beam and Focused Laser Rapid Manufacturing Methods. J Mater Eng Perform, 29:4463–73. https://doi.org/10.1007/s11665-020-04938-z.
37. Zhai W, Wang P, Ng FL, et al., 2021, Hybrid Manufacturing of γ-TiAl and Ti-6Al-4V Bimetal Component with Enhanced Strength using Electron Beam Melting. Compos B Eng, 207:108587. https://doi.org/10.1016/j.compositesb.2020.108587.
38. Wang P, Song J, Nai ML, et al., 2020, Experimental Analysis of Additively Manufactured Component and Design Guidelines for Lightweight Structures: A Case Study Using Electron Beam Melting. Addit Manuf, 33:101088. https://doi.org/10.1016/j.addma.2020.101088.
39. Zhou Q, Hayat MD, Chen G, et al., 2019, Selective Electron Beam Melting of NiTi: Microstructure, Phase Transformation and Mechanical Properties. Mater Sci Eng A, 744:290–8.
40. Thompson SM, Bian L, Shamsaei N, et al., 2015, An Overview of Direct Laser Deposition for Additive Manufacturing; Part I : Transport Phenomena, Modeling and Diagnostics. Addit Manuf, 8:36–62. https://doi.org/10.1016/j.addma.2015.07.001.
41. Lee J, Shin YC, 2019, Effects of Composition and Post Heat Treatment on Shape Memory Characteristics and Mechanical Properties for Laser Direct Deposited Nitinol. Laser Manuf Mater Process, 6:41–58. https://doi.org/10.1007/s40516-019-0079-5.
42. Yu WH, Sing SL, Chua CK, et al., 2019, Particle-Reinforced Metal Matrix Nanocomposites Fabricated by Selective Laser Melting: A State of the Art Review. Prog Mater Sci, 104:330–79. https://doi.org/10.1016/j.pmatsci.2019.04.006.
43. Lu B, Cui X, Ma W, et al., 2020, Promoting the Heterogeneous Nucleation and the Functional Properties of Directed Energy Deposited NiTi Alloy by Addition of La2O3. Addit Manuf, 33:101150. https://doi.org/10.1016/j.addma.2020.101150.
44. Saedi S, Turabi AS, Andani MT, et al., 2016, Thermomechanical Characterization of Ni-Rich NiTi Fabricated by Selective Laser Melting. Smart Mater Struct, 25:035005. https://doi.org/10.1088/0964-1726/25/3/035005.
45. Dash B, Das M, Das M, et al., 2019, A Concise Review on Machinability of NiTi Shape Memory Alloys. Mater Today, 18:5141–50. https://doi.org/10.1016/j.matpr.2019.07.511.
46. Dallago M, Fontanari V, Winiarski B, et al., 2017, Fatigue Properties of Ti6Al4V Cellular Specimens Fabricated Via SLM: CAD vs Real Geometry. Procedia Struct Integr, 7:116–23. https://doi.org/10.1016/j.prostr.2017.11.068.
47. Dadbakhsh S, Speirs M, Kruth JP, et al., 2014, Effect of SLM Parameters on Transformation Temperatures of Shape Memory Nickel Titanium Parts. Adv Eng Mater, 16:1140–6. https://doi.org/10.1002/adem.201300558.
48. Farber E, Zhu JN, Popovich A, et al., 2020, A Review of NiTi Shape Memory Alloy as a Smart Material Produced by Additive Manufacturing. Mater Today, 30:761–7. https://doi.org/10.1016/j.matpr.2020.01.563.
49. Haberland C, Frenzel J, 2012, Proceedings of the ASME Conference on Smart Materials Adaptive Structures and Intelligent Systems, September 19-21, 2012, On the Properties of Ni-Rich NiTi Shape Memory Parts Produced by Selective Laser Melting, Georgia, United States, p1–8. https://doi.org/10.1115/smasis2012-8040.
50. Biffi CA, Fiocchi J, Valenza F, et al., 2020, Selective Laser Melting of NiTi Shape Memory Alloy: Processability, Microstructure, and Superelasticity. Shap Mem Superelast, 6:342–53. https://doi.org/10.1007/s40830-020-00298-8.
51. Yang Y, Zhan JB, Sun ZZ, et al., 2019, Evolution of Functional Properties Realized by Increasing Laser Scanning Speed for the Selective Laser Melting Fabricated NiTi Alloy. J Alloys Compd, 804:220–9. https://doi.org/10.1016/j.jallcom.2019.06.340.
52. Khoo ZX, Liu Y, Low ZH, et al., 2018, Fabrication of SLM NiTi Shape Memory Alloy via Repetitive Laser Scanning. Shap Mem Superelast, 4:112–20. https://doi.org/10.1007/s40830-017-0139-7.
53. Saedi S, Saghaian SE, Jahadakbar A, et al., 2018, Shape Memory Response of Porous NiTi Shape Memory Alloys Fabricated by Selective Laser Melting. J Mater Sci Mater Med, 29:40. https://doi.org/10.1007/s10856-018-6044-6.
54. Yang Q, Kaihua S, Yang C, et al., 2020, Compression and Superelasticity Behaviors of NiTi Porous Structures with Tiny Strut Fabricated by Selective Laser Melting. J Alloys Compd, 858:157674. https://doi.org/10.1016/j.jallcom.2020.157674.
55. Fu J, Hu Z, Song X, et al., 2020, Micro Selective Laser Melting of NiTi Shape Memory Alloy: Defects, Microstructures and Thermal/Mechanical Properties. Opt Lasers Eng, 131:106374. https://doi.org/10.1016/j.optlastec.2020.106374.
56. Brailovski V, Trochu F, 1996, Review of Shape Memory Alloys Medical Applications in Russia. Biomed Mater Eng, 6:291–8. https://doi.org/10.3233/bme-1996-6406.
57. Sanders JO, Sanders AE, More R, et al., 1993, A Preliminary Investigation of Shape Memory Alloys in the Surgical Correction of Scoliosis. Spine, 18:1640–6. https://doi.org/10.1097/00007632-199309000-00012.
58. Mei FR, Ren XJ, Wang WD, 1997, The Biomechanical Effect and Clinical Application of a Ni-Ti Shape Memory Expansion Clamp. Spine, 22:2083–28. https://doi.org/10.1097/00007632-199709150-00004.
59. Nieslanik JM, 1998, Titanium-nickel shape memory clamps in small bone surgery. Arch Orthop Trauma Surg, 117:341–4. https://doi.org/10.1007/s004020050262.
60. Xu W, Frank TG, Stockham G, et al., 1999, Shape Memory Alloy Fixator System for Suturing Tissue in Minimal Access Surgery. Ann Biomed Eng, 27:663–9. https://doi.org/10.1114/1.216.
61. Kuboki Y, Jin Q, Kikuchi M, et al., 2002, Geometry of Artificial ECM: Sizes of Pores Controlling Phenotype Expression in BMP-Induced Osteogenesis and Chondrogenesis. Connect Tissue Res, 43:529–34. https://doi.org/10.1080/713713489.
62. Story BJ, Wagner WR, Gaisser DM, et al., 1998, In vivo Performance of a Modified CSTi Dental Implant Coating. Inter J Oral Maxillofac Implants, 13:749–57.
63. Rao X, Chu CL, Zheng YY, 2014, Phase Composition, Microstructure, and Mechanical Properties of Porous Ti-Nb-Zr Alloys Prepared by a Two-Step Foaming Powder Metallurgy Method. J Mech Behav Biomed Mater, 34:27–36. https://doi.org/10.1016/j.jmbbm.2014.02.001.
64. Yook SW, Do Jung H, Park CH, et al., 2012, Reverse Freeze Casting: A New Method for Fabricating Highly Porous Titanium Scaffolds with Aligned Large Pores. Acta Biomater, 8:2401–10. https://doi.org/10.1016/j.actbio.2012.03.020.
65. Karageorgiou V, Kaplan D, 2005, Porosity of 3D Biomaterial Scaffolds and Osteogenesis. Biomaterials, 26:5474–91. https://doi.org/10.1016/j.biomaterials.2005.02.002.
66. Zhang LC, Chen LY, Wang L, 2020, Surface Modification of Titanium and Titanium Alloys: Technologies, Developments, and Future Interests. Adv Eng Mater, 22:1901258. https://doi.org/10.1002/adem.201901258.
67. Wang Q, Zhou P, Liu S, et al., 2020, Multi-Scale Surface Treatments of Titanium Implants for Rapid Osseointegration: A Review. Nanomaterials, 10:1244. https://doi.org/10.3390/nano10061244.
68. Liu J, Liu J, Attarilar S, et al., 2020, Nano-Modified Titanium Implant Materials: A Way Toward Improved Antibacterial Properties. Front Bioeng Biotechnol, 8:576969. https://doi.org/10.3389/fbioe.2020.576969.
69. Lee YH, Bhattarai G, Park IS, et al., 2013, Bone Regeneration Around N-Acetyl Cysteine-Loaded Nanotube Titanium Dental Implant in Rat Mandible. Biomaterials, 34:10199–208. https://doi.org/10.1016/j.biomaterials.2013.08.080.
70. Zheng C, Attarilar S, Li K, et al., 2021, 3D-Printed HA15-Loaded β-Tricalcium Phosphate/Poly (Lactic-co-glycolic Acid) Bone Tissue Scaffold Promotes Bone Regeneration in Rabbit Radial Defects. Int J Bioprint, 7:317. https://doi.org/10.18063/ijb.v7i1.317.
71. van Bael S, Vandenbroucke B, Kerckhofs G, et al., 2009, Design and Production of Bone Scaffolds with Selective Laser Melting. In: TMS 2009 138th Annual Meeting and Exhibition, pp. 333–9. Available from: https://www.lirias. kuleuven.be/318239?limo=0.
72. van Bael S, Chai Y C, Truscello S, et al., 2012, The Effect of Pore Geometry on the In Vitro Biological Behavior of Human Periosteum-Derived Cells Seeded on Selective Laser-Melted Ti6Al4V Bone Scaffolds. Acta Biomater, 8:2824–34. https://doi.org/10.1016/j.actbio.2012.04.001.
73. Rumpler M, Woesz A, Dunlop JWC, et al., 2008, The Effect of Geometry on Three-Dimensional Tissue Growth. J R Soc Interface, 5:1173–80.
74. Li X, Tan YH, Wang P, et al., 2020, Metallic Microlattice and Epoxy Interpenetrating Phase Composites: Experimental and Simulation Studies on Superior Mechanical Properties and Their Mechanisms. Compos A Appl Sci Manuf, 135:105934. https://doi.org/10.1016/j.compositesa.2020.105934.
75. Wang P, Li X, Luo S, et al., 2021, Additively Manufactured Heterogeneously Porous Metallic Bone with Biostructural Functions and Bone-Like Mechanical Properties. J Mater Sci Technol, 62:173–9. https://doi.org/10.1016/j.jmst.2020.05.056.
76. Lin AS, Barrows TH, Cartmell SH, et al., 2003, Microarchitectural and Mechanical Characterization of Oriented Porous Polymer Scaffolds. Biomaterials, 24:481–9. https://doi.org/10.1016/s0142-9612(02)00361-7.
77. Chang BS, Lee CK, Hong KS, et al., 2000, Osteoconduction at Porous Hydroxyapatite with Various Pore Configurations. Biomaterials, 21:1291–8. https://doi.org/10.1016/s0142-9612(00)00030-2.
78. Yla HO, Ekholm C, Karlsson KH, et al., 2001, Pore Diameter of More Than 100 μm is Not Requisite for Bone Ingrowth in Rabbits. J Biomed Mater Res, 58:679–83. https://doi.org/10.1002/jbm.1069.
79. Gotz HE, Muller M, Emmel A, et al., 2004, Effect of Surface Finish on the Osseointegration of Laser-Treated Titanium Alloy Implants. Biomaterials, 25:4057–64. https://doi.org/10.1016/j.biomaterials.2003.11.002.
80. Taniguchi N, Fujibayashi S, Takemoto M, et al., 2016, Effect of Pore Size on Bone Ingrowth Into Porous Titanium Implants Fabricated by Additive Manufacturing: An In Vivo Experiment. Mater Sci Eng C Mater Biol Appl, 59:690–701. https://doi.org/10.1016/j.msec.2015.10.069.
81. Wang J, Bai F, Wang Z, et al., 2010, The Correlation Between the Internal Structure and Vascularization of Controllable Porous Bioceramic materials In Vivo: A Quantitative Study. Tissue Eng Part A, 16:12. https://doi.org/10.1089/ten.tea.2010.0148.
82. Knychala J, Bouropoulos N, Catt CJ, et al., 2013, Pore Geometry Regulates Early Stage Human Bone Marrow Cell Tissue Formation and Organisation. Ann Biomed Eng, 41:917–30. https://doi.org/10.1007/s10439-013-0748-z.
83. Bai Y, Chaudhari A, Wang H, 2020, Investigation on the Microstructure and Machinability of ASTM A131 Steel Manufactured by Directed Energy Deposition. J Mater Process Technol, 276:116410. https://doi.org/10.1016/j.jmatprotec.2019.116410.
84. Joly P, Duda GN, Schöne M, et al., 2013, Geometry-Driven Cell Organization Determines Tissue Growths in Scaffold Pores: Consequences for Fibronectin Organization. PLoS One, 8:e73545. https://doi.org/10.1371/journal.pone.0073545.
85. Hu Y, Grainger DW, Winn SR, et al., 2010, Fabrication of Poly (Alpha-Hydroxy Acid) foam Scaffolds Using Multiple Solvent Systems. J Biomed Mater Res, 59:563–72. https://doi.org/10.1002/jbm.1269.
86. Jones AC, Arns CH, Hutmacher DW, et al., 2009, The Correlation of Pore Morphology, Interconnectivity and Physical Properties of 3D Ceramic Scaffolds with Bone Ingrowth. Biomaterials, 30:1440–51. https://doi.org/10.1016/j.biomaterials.2008.10.056.
87. Wen P, Jauer L, Voshage M, et al., 2018, Densification Behavior of Pure Zn Metal Parts Produced by Selective Laser Melting for Manufacturing Biodegradable Implants. J Mater Process Technol, 258:128–37. https://doi.org/10.1016/j.jmatprotec.2018.03.007.
88. Zheng J, Chen L, Chen D, et al., 2019, Effects of Pore Size and Porosity of Surface-Modified Porous Titanium Implants on Bone Tissue Ingrowth. Trans Nonferr Metals Soc, 29:2534–45. https://doi.org/10.1016/s1003-6326(19)65161-7.
89. Takemoto M, Fujibayashi S, Matsushita T, et al., 2005, Mechanical Properties and Osteoconductivity of Porous Bioactive Titanium. Biomaterials, 26:6014–23. https://doi.org/10.1016/j.biomaterials.2005.03.019.
90. Dong XN, Guo XE, 2004, The Dependence of Transversely Isotropic Elasticity of Human Femoral Cortical Bone on Porosity. J Biomech, 37:1281–7. https://doi.org/10.1016/j.jbiomech.2003.12.011.
91. Abidi IH, Khalid FA, Farooq MU, et al., 2015, Tailoring the Pore Morphology of Porous Nitinol with Suitable Mechanical Properties for Biomedical Applications. Mater Lett, 154:17–20. https://doi.org/10.1016/j.matlet.2015.04.057.
92. Reilly GC, Engler AJ, 2010, Intrinsic Extracellular Matrix Properties Regulate Stem Cell Differentiation. J Biomech, 43:55–62. https://doi.org/10.1016/j.jbiomech.2009.09.009.
93. Chang B, Song W, Han T, et al., 2016, Influence of Pore Size of Porous Titanium Fabricated by Vacuum Diffusion Bonding of Titanium Meshes on Cell Penetration and Bone Ingrowth. Acta Biomater, 33:311–21. https://doi.org/10.1016/j.actbio.2016.01.022.
94. van Eeden SP, Ripamonti U, 1994, Bone Differentiation in Porous Hydroxyapatite in Baboons is Regulated by the Geometry of the Substratum: Implications for Reconstructive Craniofacial Surgery. Plast Reconstr Surg, 93:959–66. https://doi.org/10.1097/00006534-199404001-00010.
95. Otsuki B, Takemoto M, Fujibayashi S, et al., 2006, Pore Throat Size and Connectivity Determine Bone and Tissue Ingrowth Into Porous Implants: Three-Dimensional Micro-CT Based Structural Analyses of Porous Bioactive Titanium Implants. Biomaterials, 27:5892–900. https://doi.org/10.1016/j.biomaterials.2006.08.013.
96. Barou O, Mekraldi S, Vico L, et al., 2002, Relationships Between Trabecular Bone Remodeling and Bone Vascularization: A Quantitative Study. Bone, 30:604–12. https://doi.org/10.1016/s8756-3282(02)00677-4.
97. Chu CL, Chung CY, Lin PH, et al., 2004, Fabrication of Porous NiTi Shape Memory Alloy for Hard Tissue Implants by Combustion Synthesis. Mater Sci Eng A Struct, 366:114–9.
https://doi.org/10.1016/j.msea.2003.08.118.
98. van Oers RF, Ruimerman R, van Rietbergen B, et al., 2008, Relating Osteon Diameter to Strain. Bone, 43:476–82. https://doi.org/10.1016/j.bone.2008.05.015.
99. Tan XP, Tan YJ, Chow CS, et al., 2017, Metallic Powder-Bed Based 3D Printing of Cellular Scaffolds for Orthopaedic Implants: A State-of-the-Art Review on Manufacturing, Topological Design, Mechanical Properties and Biocompatibility. Mater Sci Eng C Mater Biol Appl, 76:1328–43. https://doi.org/10.1016/j.msec.2017.02.094.
100. Soro N, Attar H, Wu X, et al., 2019, Investigation of the Structure and Mechanical Properties of Additively Manufactured Ti-6Al-4V Biomedical Scaffolds Designed with a Schwartz Primitive Unit-Cell. Mater Sci Eng A Struct, 745:195–202. https://doi.org/10.1016/j.msea.2018.12.104.
101. Attar H, Löber L, Funk A, et al., 2015, Mechanical Behavior of Porous Commercially Pure Ti and Ti-TiB Composite Materials Manufactured by Selective Laser Melting. Mater Sci Eng A Struct, 625:350–6. https://doi.org/10.1016/j.msea.2014.12.036.
102. Soro N, Attar H, Brodie E, et al., 2019, Evaluation of the Mechanical Compatibility of Additively Manufactured Porous Ti-25Ta Alloy for Load-Bearing Implant Applications. J Mech Behav Biomed, 97:149–58. https://doi.org/10.1016/j.jmbbm.2019.05.019.
103. Lowther M, Louth S, Davey A, et al., 2019, Clinical, Industrial, and Research Perspectives on Powder Bed Fusion Additively Manufactured Metal Implants. Addit Manuf, 28:565–84. https://doi.org/10.1016/j.addma.2019.05.033.
104. Attar H, Ehtemam-Haghighi S, Soro N, et al., 2020, Additive Manufacturing of Low-Cost Porous Titanium-Based Composites for Biomedical Applications: Advantages, Challenges and Opinion for Future Development. J Alloys Compd, 827:154263. https://doi.org/10.1016/j.jallcom.2020.154263.
105. Elahinia M, Shayesteh N, Taheri M, et al., 2016, Progress in Materials Science Fabrication of NiTi through Additive Manufacturing: A Review. Prog Mater Sci, 83:630–63. https://doi.org/10.1016/j.pmatsci.2016.08.001.
106. Shishkovsky I, Yadroitsev I, Smurov I, 2012, Direct Selective Laser Melting of Nitinol Powder. Phys Procedia, 39:447–54. https://doi.org/10.1016/j.phpro.2012.10.060.
107. Shiva S, Palani IA, Mishra SK, et al., 2015, Investigations on the Influence of Composition in the Development of Ni-Ti Shape Memory Alloy using Laser Based Additive Manufacturing. Opt Laser Technol, 69:44–51. https://doi.org/10.1016/j.optlastec.2014.12.014.
108. Yang Y, Zhan JB, Sui JB, et al., 2020, Functionally Graded NiTi Alloy with Exceptional Strain-Hardening Effect Fabricated by SLM Method. Scr Mater, 188:130–4. https://doi.org/10.1016/j.scriptamat.2020.07.019.
109. Saedi S, Turabi A S, Andani MT, et al., 2016, The Influence of Heat Treatment on the Thermomechanical Response of Ni-Rich NiTi Alloys Manufactured by Selective Laser Melting. J Alloys Compd, 677:204–10. https://doi.org/10.1016/j.jallcom.2016.03.161.
110. Imran M, Zhang X, 2020, Recent Developments on the Cyclic Stability in Elastocaloric Materials. Mater Des, 195:109030. https://doi.org/10.1016/j.matdes.2020.109030.
111. Dadbakhsh S, Speirs M, Kruth JP, et al., 2015, Influence of SLM on Shape Memory and Compression Behaviour of NiTi Scaffolds. CIRP Ann Manuf Technol, 64(1):209–12. https://doi.org/10.1016/j.cirp.2015.04.039.
112. Bormann T, Schulz G, Deyhle H, et al., 2014, Combining Micro Computed Tomography and Three-Dimensional Registration to Evaluate Local Strains in Shape Memory Scaffolds. Acta Biomater, 10:1024–34. https://doi.org/10.1016/j.actbio.2013.11.007.
113. Andani MT, Saedi S, Turabi AS, et al., 2017, Mechanical and Shape Memory Properties of Porous Ni50.1Ti49.9 Alloys Manufactured by Selective Laser Melting. J Mech Behav Biomed, 68:224–31. https://doi.org/10.1016/j.jmbbm.2017.01.047.
114. Ma C, Gu D, Lin K, et al., 2019, Selective Laser Melting Additive Manufacturing of Cancer pagurus’s Claw Inspired Bionic Structures with High Strength and Toughness. Appl Surf Sci, 469:647–56. https://doi.org/10.1016/j.apsusc.2018.11.026.
115. Xiong Z, Li Z, Sun Z, et al., 2019, Selective Laser Melting of NiTi Alloy with Superior Tensile Property and Shape Memory Effect. J Mater Sci Technol, 35:2238–42. https://doi.org/10.1016/j.jmst.2019.05.015.
116. Yang KV, Rometsch P, Jarvis T, et al., 2018, Porosity Formation Mechanisms and Fatigue Response in Al-Si-Mg Alloys Made by Selective Laser Melting. Mater Sci Eng A Struct, 712:166–74. https://doi.org/10.1016/j.msea.2017.11.078.
117. Prashanth KG, Scudino S, Eckert J, 2017, Defining the Tensile Properties of Al-12Si Parts Produced by Selective Laser Melting. Acta Mater, 126:25–35. https://doi.org/10.1016/j.actamat.2016.12.044.
118. Zhou X, Li K, Zhang D, et al., 2015, Textures Formed in a CoCrMo Alloy by Selective Laser Melting. J Alloys Compd, 631:153–64.
119. Wang X, Yu J, Liu J, et al., 2020, Effect of Process Parameters on the Phase Transformation Behavior and Tensile Properties of NiTi Shape Memory Alloys Fabricated by Selective Laser Melting. Addit Manuf, 36:101545. https://doi.org/10.1016/j.addma.2020.101545.
120. Ou SF, Peng BY, Chen YC, et al., 2018, Manufacturing and Characterization of NiTi Alloy with Functional Properties by Selective Laser Melting. Metals, 8:342. https://doi.org/10.3390/met8050342.
121. Kaur M, Singh K, 2019, Review on Titanium and Titanium Based Alloys as Biomaterials for Orthopaedic Applications. Mater Sci Eng C, 102:844–62.
122. Moghaddam NS, Saghaian SE, Amerinatanzi A, et al., 2018, Anisotropic Tensile and Actuation Properties of NiTi Fabricated with Selective Laser Melting. Mater Sci Eng A Struct, 724:220–30. https://doi.org/10.1016/j.msea.2018.03.072.
123. Bayati P, Jahadakbar A, Barati M, et al., 2020, Toward Low and High Cycle Fatigue Behavior of SLM-Fabricated NiTi: Considering the Effect of Build Orientation and Employing a Self-Heating Approach. Int J Mech Sci, 185:105878. https://doi.org/10.1016/j.ijmecsci.2020.105878.
124. Zhang Q, Hao S, Liu Y, et al., 2020, The Microstructure of a Selective Laser Melting (SLM)-Fabricated NiTi Shape Memory Alloy with Superior Tensile Property and Shape Memory Recoverability. Appl Mater Today, 19:100547. https://doi.org/10.1016/j.apmt.2019.100547.
125. Koike M, Martinez K, Guo L, et al., 2011, Evaluation of Titanium Alloy Fabricated using Electron Beam Melting System for Dental Applications. J Mater Process Technol, 211:1400–8.
126. Speirs M, van Hooreweder B, van Humbeeck J, et al., 2017, Fatigue Behaviour of NiTi Shape Memory Alloy Scaffolds Produced by SLM, a Unit Cell Design Comparison. J Mech Behav Biomed, 70:53–59. https://doi.org/10.1016/j.jmbbm.2017.01.016.
127. Dallago M, Zanini F, Carmignato S, et al., 2018, Effect of the Geometrical Defectiveness on the Mechanical Properties of SLM Biomedical Ti6Al4V Lattices. Procedia Struct Integr, 13:161–7. https://doi.org/10.1016/j.prostr.2018.12.027.
128. de Wild M, Meier F, Bormann T, et al., 2014, Damping of Selective-Laser-Melted NiTi for Medical Implants. J Mater Eng Perform, 23:2614–9. https://doi.org/10.1007/s11665-014-0889-8.
129. Attarilar S, Salehi MT, Al-Fadhalah KJ, et al., 2019, Functionally Graded Titanium Implants: Characteristic Enhancement Induced by Combined Severe Plastic Deformation. PLoS One, 14:1–18. https://doi.org/10.1371/journal.pone.0221491.
130. Alves AC, Thibeaux R, Toptan F, et al., 2019, Influence of Macroporosity on NIH/3T3 Adhesion, Proliferation, and Osteogenic Differentiation of MC3T3-E1 Over Bio-Functionalized Highly Porous Titanium Implant Material. J Biomed Mater Res B, 107:73–85. https://doi.org/10.1002/jbm.b.34096.
131. Hafeez N, Liu J, Wang L, et al., 2020, Superelastic Response of Low-Modulus Porous Beta-Type Ti-35Nb-2Ta-3Zr Alloy Fabricated by Laser Powder Bed Fusion. Addit Manuf, 34:101264. https://doi.org/10.1016/j.addma.2020.101264.
132. Liu R, Liu S, Zhang X, 2021, A Physics-Informed Machine Learning Model for Porosity Analysis in Laser Powder Bed Fusion Additive Manufacturing. Int J Adv Manuf Tech, 113:1943–58. https://doi.org/10.1007/s00170-021-06640-3.
133. Wang C, Tan XP, Tor SB, et al., 2020, Machine Learning in Additive Manufacturing: State-of-the-Art and Perspectives. Addit Manuf, 36:101538. https://doi.org/10.1016/j.addma.2020.101538.
134. Ng WL, Chan A, Ong YS, et al., 2020, Deep Learning for Fabrication and Maturation of 3D Bioprinted Tissues and Organs. Virtual Phys Prototyp, 15:340–58.
135. Goh GD, Sing SL, Yeong WY, 2020, A Review on Machine Learning in 3D Printing: Applications, Potential, and Challenges. Artif Intell Rev, 54:63–94. https://doi.org/10.1007/s10462-020-09876-9.