Optimizing implant lattice design for large distal femur defects: Stimulating interface bone growth to enhance osseointegration
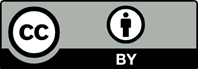
Large bone defects in the distal femur present a significant challenge due to the lack of inherent self-healing capabilities. Traditional approaches, such as utilizing polymethyl methacrylate (PMMA) in conjunction with a plate for distal femur reconstruction, have shown unsatisfactory osseointegration outcome, which leads to complications. To address this challenge, this study focuses on developing a lattice-structured implant for reconstructing distal femoral bone defects. The lattice geometry is based on the cuboctahedron lattice, with its design optimized through the adjustment of pillar diameter and arrangement angle. The lattice structure is designed to stimulate the surrounding bone, ultimately enhancing osseointegration in distal femur reconstruction. Finite element analysis revealed that for promoting bone ingrowth toward the implant, setting the optimal lattice structure parameters, i.e., a 45° arrangement angle and a 0.8 mm pillar diameter, is required. Fabricated using state-of-the-art metal three-dimensional printing, the implant underwent rigorous validation through biomechanical testing, in vitro biological assays, and animal experiments. The comprehensive results affirmed the bioactivity of the lattice-structured implant, underscoring its capability to improve osseointegration in distal femoral defect reconstruction.
- Wiese A, Pape HC. Bone defects caused by high-energy injuries, bone loss, infected nonunions, and nonunions. Orthop Clin North Am. 2010;41(1):1-4. doi: 10.1016/j.ocl.2009.07.003
- Seitz H, Rieder W, Irsen S, Leukers B, Tille C. Three-dimensional printing of porous ceramic scaffolds for bone tissue engineering. J Biomed Mater Res B Appl Biomater. 2005;74(4):782-788. doi: 10.1002/jbm.b.30291
- Jones AC, Arns CH, Sheppard AP, Hutmacher DW, Milthorpe BK, Knackstedt MA. Assessment of bone ingrowth into porous biomaterials using MICRO-CT. Biomaterials. 2007;28(15):2491-2504. doi: 10.1016/j.biomaterials.2007.01.046
- Eil Bakhtiari SS, Bakhsheshi-Rad HR, Karbasi S, et al. Polymethyl methacrylate-based bone cements containing carbon nanotubes and graphene oxide: an overview of physical, mechanical, and biological properties. Polymers. 2020;12(7):1469. doi: 10.3390/polym12071469
- Vaishya R, Chauhan M, Vaish A. Bone cement. J Clin Orthop Trauma. 2013;4(4):157-163. doi: 10.1016/j.jcot.2013.11.005
- Gundapaneni D, Goswami T. Thermal isotherms in PMMA and cell necrosis during total hip arthroplasty. J Appl Biomater Funct Mater. 2014;12(3):193-202. doi: 10.5301/jabfm.5000196
- Revie IC, Wallace ME, Orr JF. The effect of PMMA thickness on thermal bone necrosis around acetabular sockets. Proc Inst Mech Eng H. 1994;208(1):45-51. doi: 10.1177/095441199420800106
- Hasandoost L, Rodriguez O, Alhalawani A, et al. The role of poly(methyl methacrylate) in management of bone loss and infection in revision total knee arthroplasty: a review. J Funct Biomater. 2020;11(2):25. doi: 10.3390/jfb11020025
- Mnaymneh W, Malinin TI, Lackman RD, Hornicek FJ, Ghandur-Mnaymneh L. Massive distal femoral osteoarticular allografts after resection of bone tumors. Clin Orthop Relat Res. 1994;303:103-115. doi: 10.1097/00003086-199406000-00013
- Kim T, See CW, Li X, Zhu D. Orthopedic implants and devices for bone fractures and defects: past, present and perspective. Eng Regen. 2020;1:6-18. doi: 10.1016/j.engreg.2020.05.003
- Song Y, Xu DS, Yang R, Li D, Wu WT, Guo ZX. Theoretical study of the effects of alloying elements on the strength and modulus of β-type bio-titanium alloys. Mater Sci Eng A. 1999;260(1):269-274. doi: 10.1016/S0921-5093(98)00886-7
- Velasco MA, Narváez-Tovar CA, Garzón-Alvarado DA. Design, materials, and mechanobiology of biodegradable scaffolds for bone tissue engineering. Biomed Res Int. 2015;2015:729076. doi: 10.1155/2015/729076
- Geetha M, Singh AK, Asokamani R, Gogia AK. Ti based biomaterials, the ultimate choice for orthopaedic implants – a review. Prog Mater Sci. 2009;54(3):397-425. doi: 10.1016/j.pmatsci.2008.06.004
- Liu Y, Li K, Wu H, et al. Synthesis of Ti-Ta alloys with dual structure by incomplete diffusion between elemental powders. J Mech Behav Biomed Mater. 2015;51:302-312. doi: 10.1016/j.jmbbm.2015.07.004
- Arabnejad S, Burnett Johnston R, Pura JA, Singh B, Tanzer M, Pasini D. High-strength porous biomaterials for bone replacement: a strategy to assess the interplay between cell morphology, mechanical properties, bone ingrowth and manufacturing constraints. Acta Biomater. 2016;30:345-356. doi: 10.1016/j.actbio.2015.10.048
- Hsieh MT, Begley MR, Valdevit L. Architected implant designs for long bones: advantages of minimal surface-based topologies. Mater Des. 2021;207:109838. doi: 10.1016/j.matdes.2021.109838
- Mikuni-Takagaki Y, Suzuki Y, Kawase T, Saito S. Distinct responses of different populations of bone cells to mechanical stress. Endocrinology. 1996;137(5):2028-2035. doi: 10.1210/endo.137.5.8612544
- Ehrlich PJ, Lanyon LE. Mechanical strain and bone cell function: a review. Osteoporos Int. 2002;13(9):688-700. doi: 10.1007/s001980200095
- Al-Tamimi AA, Almeida H, Bartolo P. Structural optimization for medical implants through additive manufacturing. Prog Addit Manuf. 2020;5(2):95-110. doi: 10.1007/s40964-020-00109-7
- Burton HE, Eisenstein NM, Lawless BM, et al. The design of additively manufactured lattices to increase the functionality of medical implants. Mater Sci Eng C Mater Biol Appl. 2019;94:901-908. doi: 10.1016/j.msec.2018.10.052
- Wang P, Li X, Luo S, Nai MLS, Ding J, Wei J. Additively manufactured heterogeneously porous metallic bone with biostructural functions and bone-like mechanical properties. J Mater Sci Technol. 2021;62:173-179. doi: 10.1016/j.jmst.2020.05.056
- Bucklen BS, Wettergreen WA, Yuksel E, Liebschner MAK. Bone-derived CAD library for assembly of scaffolds in computer-aided tissue engineering. Virtual Phys Prototyp. 2008;3(1):13-23. doi: 10.1080/17452750801911352
- Van Bael S, Chai YC, Truscello S, et al. The effect of pore geometry on the in vitro biological behavior of human periosteum-derived cells seeded on selective laser-melted Ti6Al4V bone scaffolds. Acta Biomater. 2012;8(7):2824- 2834. doi: 10.1016/j.actbio.2012.04.001
- Guoqing Z, Junxin L, Chengguang Z, Juanjuan X, Xiaoyu Z, Anmin W. Design optimization and manufacturing of bio-fixed tibial implants using 3D printing technology. J Mech Behav Biomed Mater. 2021;117:104415. doi: 10.1016/j.jmbbm.2021.104415
- Mishra RN, Singh MK, Kumar V. Biomechanical analysis of human femur using finite element method: a review study. Mater Today: Proc. 2022;56(1):384-389. doi: 10.1016/j.matpr.2022.01.222
- Alabort E, Barba D, Reed RC. Design of metallic bone by additive manufacturing. Scr Mater. 2019;164:110-114. doi: 10.1016/j.scriptamat.2019.01.022
- Lozanovski B, Leary M, Tran P, et al. Computational modelling of strut defects in SLM manufactured lattice structures. Mater Des. 2019;171:107671. doi: 10.1016/j.matdes.2019.107671
- Ejnisman L, Gobbato B, de França Camargo AF, Zancul E. Three-dimensional printing in orthopedics: from the basics to surgical applications. Curr Rev Musculoskelet Med. 2021;14(1):1-8. doi: 10.1007/s12178-020-09691-3
- Wang YT, Hsu CK. Novel parameter optimization lattice design for improving osseointegration in hypo-loading regions – a case study of maxillary tumor reconstruction implant. Mater Des. 2023;233(2023):112274. doi: 10.1016/j.matdes.2023.112274
- Lee KS, Lee WC, Kim PG, et al. Biomechanical evaluation of initial stability of a root analogue implant design withdrilling protocol: a 3D finite element analysis. Appl Sci. 2020;10:4104. doi: 10.3390/app10124104
- Delikanli YE, Kayacan MC. Design, manufacture, and fatigue analysis of lightweight hip implants. J Appl Biomater Funct Mater. 2019;17(2):2280800019836830. doi: 10.1177/2280800019836830
- Kang J, Dong E, Li X, et al. Topological design and biomechanical evaluation for 3D printed multi-segment artificial vertebral implants. Biomater Adv. 2021;127: 112250. doi: 10.1016/j.msec.2021.112250
- Kang H, Lin CY, Hollister SJ. Topology optimization of three dimensional tissue engineering scaffold architectures for prescribed bulk modulus and diffusivity. Struct Multidiscipl Optim. 2010;42(4):633-644. doi: 10.1007/s00158-010-0508-8
- Rayhan SB, Rahman M. Modeling elastic properties of unidirectional composite materials using Ansys material designer. Int J Struct Integr. 2020;28:1892-1900. doi: 10.1016/j.prostr.2020.11.012
- Raja BK, Arun KC, Dheenadayalan J. Classification of distal femur fractures and their clinical relevance. Trauma Int. 2016;2(1):3-6. doi: 10.13107/ti.2016.v02i01.012
- Schmidt U, Penzkofer R, Bachmaier S, Augat P. Implant material and design alter construct stiffness in distal femur locking plate fixation: a pilot study. Clin Orthop Relat Res. 2013;471(9):2808-2814. doi: 10.1007/s11999-013-2867-0
- Ahirwar H, Gupta VK, Nanda HS. Finite element analysis of fixed bone plates over fractured femur model. Comput Methods Biomech Biomed Engin. 2021;24(15): 1742-1751. doi: 10.1080/10255842.2021.1918123
- Chethan KN, Bhat SN, Shenoy SB. Biomechanics of hip joint: a systematic review. Int J Eng Technol. 2018;7(3): 1672-1676. doi: 10.14419/ijet.v7i3.15231
- Darwish SM, Al-Samhan AM. Optimization of artificial hip joint parameters. Materwiss Werksttech. 2009;40(3):218-223. doi: 10.1002/mawe.200900430
- Niinomi M. Mechanical properties of biomedical titanium alloys. Mater Sci Eng A. 1998;243(1):231-236. doi: 10.1016/S0921-5093(97)00806-X
- Reina-Romo E, Sampietro-Fuentes A, Gómez-Benito MJ, Domínguez J, Doblaré M, García-Aznar JM. Biomechanical response of a mandible in a patient affected with hemifacial microsomia before and after distraction osteogenesis. Med Eng Phys. 2010;32(8):860-866. doi: 10.1016/j.medengphy.2010.05.012
- Zhang Y, Yan C, Zhang L, Zhang W, Wang G. Comparison of ordinary cannulated compression screw and double-head cannulated compression screw fixation in vertical femoral neck fractures. Biomed Res Int. 2020;2020:2814548. doi: 10.1155/2020/2814548
- Maia PW, Teixeira ML, Scavone de Macedo LG, et al. Use of platelet-rich fibrin associated with xenograft in critical bone defects: histomorphometric study in rabbits. Symmetry. 2019;11(10):1293. doi: 10.3390/sym11101293
- Bai MY, Wang CW, Wang JY, Lin MF, Chan WP. Three-dimensional structure and cytokine distribution of platelet-rich fibrin. Clinics (Sao Paulo). 2017;72(2):116-124. doi: 10.6061/clinics/2017(02)09
- Wu PK, Lee CW, Sun WH, Lin CL. Biomechanical analysis and design method for patient-specific reconstructive implants for large bone defects of the distal lateral femur. Biosensors. 2022;12(1):4. doi: 10.3390/bios12010004
- Bergmann G, Deuretzbacher G, Heller M, et al. Hip contact forces and gait patterns from routine activities. J Biomech. 2001;34(7):859-871. doi: 10.1016/s0021-9290(01)00040-9
- Callaghan JP, McGill SM. Low back joint loading and kinematics during standing and unsupported sitting. Ergonomics. 2001;44(3):280-294. doi: 10.1080/00140130118276
- Hamandi F, Laughlin R, Goswami T. Failure analysis of PHILOS plate construct used for pantalar arthrodesis paper II—screws and FEM simulations. Metals. 2018;8(4):279. doi: 10.3390/met8040279
- Arabnejad S, Burnett Johnston R, Pura JA, Singh B, Tanzer M, Pasini D. High-strength porous biomaterials for bone replacement: a strategy to assess the interplay between cell morphology, mechanical properties, bone ingrowth and manufacturing constraints. Acta Biomater. 2016;30: 345-356. doi: 10.1016/j.actbio.2015.10.048
- Park JY, Park SH, Kim MG, Park SH, Yoo TH, Kim MS. Biomimetic scaffolds for bone tissue engineering. Adv Exp Med Biol. 2018;1064:109-121. doi: 10.1007/978-981-13-0445-3_7
- Boyle C, Kim IY. Comparison of different hip prosthesis shapes considering micro-level bone remodeling and stress-shielding criteria using three-dimensional design space topology optimization. J Biomech. 2011;44(9):1722-1728. doi: 10.1016/j.jbiomech.2011.03.038
- Rahmanian R, Moghaddam NS, Haberland C, Dean D, Miller M, Elahinia M. Load bearing and stiffness tailored NiTi implants produced by additive manufacturing: a simulation study. Proc SPIE. 2014;9058:905814. doi: 10.1117/12.2048948
- Alghamdi HS. Methods to improve osseointegration of dental implants in low quality (type-IV) bone: an overview. J Funct Biomater. 2018;9(1):7. doi: 10.3390/jfb9010007
- Maskery I, Aremu AO, Parry L, Wildman RD, Tuck CJ, Ashcroft IA. Effective design and simulation of surface-based lattice structures featuring volume fraction and cell type grading. Mater Des. 2018;155:220-232. doi: 10.1016/j.matdes.2018.05.058
- Sahu NK, Andhare AB. Multiobjective optimization for improving machinability of Ti-6Al-4V using RSM and advanced algorithms. J Comput Des Eng. 2019;6(1):1-12. doi: 10.1016/j.jcde.2018.04.004
- Taniguchi N, Fujibayashi S, Takemoto M, et al. Effect of pore size on bone ingrowth into porous titanium implants fabricated by additive manufacturing: an in vivo experiment. Mater Sci Eng C Mater Biol Appl. 2016;59:690-701. doi: 10.1016/j.jmst.2020.05.056
- Roberts TT, Rosenbaum AJ. Bone grafts, bone substitutes and orthobiologics: the bridge between basic science and clinical advancements in fracture healing. Organogenesis. 2012;8(4):114-124. doi: 10.4161/org.23306
- Bhatt RA, Rozental TD. Bone graft substitutes. Hand Clin. 2012;28(4):457-468. doi: 10.1016/j.hcl.2012.08.001
- Harwood PJ, Ferguson DO, Michael ALR. An update on fracture healing and non-union. Orthop Trauma. 2010;24(1):9-23. doi: 10.1016/j.mporth.2009.12.004
- Fisher DM, Wong JM, Crowley C, Khan WS. Preclinical and clinical studies on the use of growth factors for bone repair: a systematic review. Curr Stem Cell Res Ther. 2013;8(3):260-268. doi: 10.2174/1574888x11308030011
- Kharmanda G. Integration of multi-objective structural optimization into cementless hip prosthesis design: improved Austin-Moore model. Comput Methods Biomech Biomed Engin. 2016;19(14):1557-1566. doi: 10.1080/10255842.2016.1170121
- Xiong YZ, Gao RN, Zhang H, Dong LL, Li JT, Li X. Rationally designed functionally graded porous Ti6Al4V scaffolds with high strength and toughness built via selective laser melting for load-bearing orthopedic applications. J Mech Behav Biomed Mater. 2020;104:103673. doi: 10.1016/j.jmbbm.2020.103673
- Ding W. Opportunities and challenges for the biodegradable magnesium alloys as next-generation biomaterials. Regen Biomater. 2016;3(2):79-86. doi: 10.1093/rb/rbw003