Computational fluid dynamics for the optimization of internal bioprinting parameters and mixing conditions
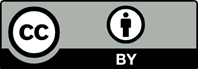
Tissue engineering requires the fabrication of three-dimensional (3D) multimaterial structures in complex geometries mimicking the hierarchical structure of biological tissues. To increase the mechanical and biological integrity of the tissue engineered structures, continuous printing of multiple materials through a printing head consisting of a single nozzle is crucial. In this work, numerical analysis was carried out to investigate the extrusion process of two different shear-thinning biomaterial solutions (alginate and gelatin) inside a novel single-nozzle dispensing system consisting of cartridges and a static mixer for varying input pressures, needle geometries, and outlet diameters. Systematic analysis of the dispensing process was conducted to describe the flow rate, velocity field, pressure drop, and shear stress distribution throughout the printing head. The spatial distribution of the biopolymer solutions along the mixing chamber was quantitatively analyzed and the simulation results were validated by comparing the pressure drop values with empirical correlations. The simulation results showed that the proposed dispensing system enables to fabricate homogenous material distribution across the nozzle outlet. The predicted shear stress along the proposed printing head model is lower than the critical shear values which correspond to negligible cell damage, suggesting that the proposed dispensing system can be used to print cell-laden tissue engineering constructs.
- Emmermacher J, Spura D, Cziommer J, et al., 2020, Engineering considerations on extrusion-based bioprinting: Interactions of material behavior, mechanical forces and cells in the printing needle. Biofabrication, 12(2):2–16. https://doi.org/10.1088/1758-5090/ab7553
- Jiang T, Munguia-Lopez JG, Flores-Torres S, et al., 2019, Extrusion bioprinting of soft materials: An emerging technique for biological model fabrication. Appl Phys Rev, 6(1). https://doi.org/10.1063/1.5059393
- Trujillo-De Santiago G, Alvarez M, Samandari M, et al., 2018, Chaotic printing: Using chaos to fabricate densely packed micro- and nanostructures at high resolution and speed. Mater Horizons, 5(5):813–822. https://doi.org/10.1039/c8mh00344k
- Gungor-Ozkerim PS, Inci I, Zhang YS, et al., 2018, Bioinks for 3D bioprinting: An overview. Biomater Sci, 6(6):915–946. https://doi.org/10.1039/c7bm00765e
- Bartolo P, Malshe A, Ferraris E, et al., 2022, 3D bioprinting: Materials, processes, and applications. CIRP Ann, 71(2): 577–597. https://doi.org/10.1016/j.cirp.2022.06.001
- Liu F, Vyas C, Yang J, et al., 2021, A review of hybrid biomanufacturing systems applied in tissue regeneration, in Virtual Prototyping & Bio Manufacturing in Medical Applications, 187–213. https://doi.org/10.1007/978-3-030-35880-8_8
- Li X, Liu B, Pei B, et al., 2020, Inkjet bioprinting of biomaterials. Chem Rev, 120(19):10793–10833. https://doi.org/10.1021/acs.chemrev.0c00008
- Ng WL, Xi H, Shkolnikov V, et al., 2021, Controlling droplet impact velocity and droplet volume: Key factors to achieving high cell viability in sub-nonoliter droplet-based bioprinting. Int J Bioprint, 8(1):424. https://doi.org/10.18063/IJB.V8I1.424
- Zhuang P, Ng WL, An J, et al., 2019, Layer-by-layer ultraviolet assisted extrusion-based (UAE) bioprinting of hydrogel constructs with high aspect ratio for soft tissue engineering applications. PLoS One, 14(6):e0216776. https://doi.org/10.1371/journal.pone.0216776
- Ng WL, Lee JM, Zhou M, et al., 2020, Vat polymerization-based bioprinting-process, materials, applications and regulatory challenges. Biofabrication, 12(2):022001. https://doi.org/10.1088/1758-5090/ab6034
- Li W, Mille LS, Robledo JA, et al., 2020, Recent advances in formulating and processing biomaterial inks for vat polymerization-based 3D printing. Adv Healthc Mater, 9(15):e2000156. https://doi.org/10.1002/adhm.202000156
- Ning L, Chen X, 2017, A brief review of extrusion-based tissue scaffold bio-printing. Biotechnol J, 12(8). https://doi.org/10.1002/biot.201600671
- Ozbolat IT, Hospodiuk M, 2016, Current advances and future perspectives in extrusion-based bioprinting. Biomaterials, 76:321–343. https://doi.org/10.1016/j.biomaterials.2015.10.076
- Kang D, Hong G, An S, et al., 2020, Bioprinting of multiscaled hepatic lobules within a highly vascularized construct. Small, 16(13):1–9. https://doi.org/10.1002/smll.201905505
- Chávez-Madero C, Derby MD, Samandari M, et al., 2020, Using chaotic advection for facile high-throughput fabrication of ordered multilayer micro-and nanostructures: Continuous chaotic printing. Biofabrication, 12(3). https://doi.org/10.1088/1758-5090/ab84cc
- Ravanbakhsh H, Karamzadeh V, Bao G, et al., 2021, Emerging technologies in multi-material bioprinting. Adv Mater, 33(49). https://doi.org/10.1002/adma.202104730
- Costantini M, Testa S, Mozetic P, et al., 2017, Microfluidic-enhanced 3D bioprinting of aligned myoblast-laden hydrogels leads to functionally organized myofibers in vitro and in vivo. Biomaterials, 131:98–110. https://doi.org/10.1016/j.biomaterials.2017.03.026
- Prendergast ME, Burdick JA, 2020, Recent advances in enabling technologies in 3D printing for precision medicine. Adv Mater, 32(13). https://doi.org/10.1002/adma.201902516
- Sodupe-Ortega E, Sanz-Garcia A, Pernia-Espinoza A, et al., 2018, Accurate calibration in multi-material 3D bioprinting for tissue engineering. Materials (Basel), 11(8):1–19. https://doi.org/10.3390/ma11081402
- Kolesky DB, Truby RL, Gladman AS, et al.,2014, 3D bioprinting of vascularized, heterogeneous cell-laden tissue constructs. Adv Mater, 26(19):3124–3130. https://doi.org/10.1002/adma.201305506
- Nadernezhad A, Khani N, Skvortsov G, et al., 2016, Multifunctional 3D printing of heterogeneous hydrogel structures. Sci Rep, 6. https://doi.org/10.1038/srep33178
- Liu W, Zhang YS, Heinrich MA, et al., 2017, Rapid continuous multimaterial extrusion bioprinting. Adv Mater, 29(3):1–8. https://doi.org/10.1002/adma.201604630
- Colosi C, Shin SR, Manoharan V, et al., 2016, Microfluidic bioprinting of heterogeneous 3D tissue constructs using low-viscosity bioink. Adv Mater, 28(4):677–684a. https://doi.org/10.3390/MI11050459
- Wang J, Zhang N, Chen J, et al., 2019, Finding the optimal design of a passive microfluidic mixer. Lab Chip, 19(21):3618–3627. https://doi.org/10.1039/c9lc00546c
- Ceballos-González CF, Bolívar-Monsalve EJ, Quevedo- Moreno DA, et al., 2021, High-throughput and continuous chaotic bioprinting of spatially controlled bacterial microcosms. ACS Biomater Sci Eng, 7(6):2408–2419. https://doi.org/10.1021/acsbiomaterials.0c01646
- Li M, Tian X, Schreyer DJ, et al., 2011, Effect of needle geometry on flow rate and cell damage in the dispensing-based biofabrication process. Biotechnol Prog, 27(6):1777–1784. https://doi.org/10.1002/btpr.679
- Andrew CD, Susan EC, Emily MR., et al., 2016, A comparison of different bioinks for 3D bioprinting of fibrocartilage and hyaline cartilage. Biofabrication, 8(4):45002. https://doi.org/10.1088/1758-5090/8/4/045002
- Samandari M, Alipanah F, Majidzadeh AK, et al., 2021, Controlling cellular organization in bioprinting thrountalizationgh designed 3D microcompartme. Appl Phys Rev, 8(2). https://doi.org/10.1063/5.0040732
- Yu Y, Zhang Y, Martin JA, et al., 2013, Evaluation of cell viability and functionality in vessel-like bioprintable cell-laden tubular channels. J Biomech Eng, 135(9):1–9. https://doi.org/10.1115/1.4024575
- Li M, Tian X, Zhu N, 2010, Modeling process-induced cell damagein the biodispensing process. Tissue Eng Part C: Methods, 16(3):533–542. https://doi.org/10.1089=ten.tec.2009.0178533
- Chiesa I, Ligorio C, Bonatti AF, et al., 2020, Modeling the three-dimensional bioprinting process of β-sheet self-assembling peptide hydrogel scaffolds. Front Med Technol, 2:1–16. https://doi.org/10.3389/fmedt.2020.571626
- Müller M, Öztürk E, Arlov Ø, et al., 2017, Alginate sulfate– nanocellulose bioinks for cartilage bioprinting applications. Ann Biomed Eng, 45(1):210–223. https://doi.org/10.1007/s10439-016-1704-5
- Serna JA, Florez SL, Talero VA, et al., 2019, Formulation and characterization of a SIS-based photocrosslinkable bioink. Polymers (Basel), 11(3):1–10. https://doi.org/10.3390/polym11030569
- Billiet T, Gevaert E, De Schryver T, et al., 2014, The 3D printing of gelatin methacrylamide cell-laden tissue-engineered constructs with high cell viability. Biomaterials, 35(1):49–62. https://doi.org/10.1016/j.biomaterials.2013.09.078
- Mahammedi A, Ameur H, Ariss A, et al., 2017, Numerical investigation of the performance of Kenics static mixers for the agitation of shear thinning fluids. J Appl Fluid Mech, 10(3):989–999. https://doi.org/10.18869/acadpub.jafm.73.240.27314
- Hobbs DM, Swanson PD, Muzzio FJ, 1998, Numerical characterization of low Reynolds number flow in the Kenics static mixer. Chem Eng Sci, 53(8):1565–1584. https://doi.org/10.1016/S0009-2509(97)00132-2
- Nyande BW, Mathew Thomas K, Lakerveld R, 2021, CFD analysis of a Kenics static mixer with a low pressure drop under laminar flow conditions. Ind Eng Chem Res, 60(14):5264–5277. https://doi.org/10.1021/acs.iecr.1c00135
- Bird RB, 2007, Transport Phenomena Rev, 2nd ed, New York, Wiley.
- Chhabra RP, Richardson JF, 2011, Chapter 1. Non- Newtonian fluid behaviour, in Non-Newtonian Flow and Applied Rheology: Engineering Applications, 536. https://doi.org/10.1016/b978-075063770-1/50002-6
- Neofytou P, 2005, A 3rd order upwind finite volume method for generalised Newtonian fluid flows. Adv Eng Softw, 36(10):664–680. https://doi.org/10.1016/j.advengsoft.2005.03.011
- Metzner AB, 1957, Non-Newtonian fluid flow relationships between recent pressure-drop correlations. Ind Eng Chem, 49(9):1429–1432. https://doi.org/10.1021/ie50573a049
- Blaeser A, Million N, Campos DFD, et al., 2016, Laser-based in situ embedding of metal nanoparticles into bioextruded alginate hydrogel tubes enhances human endothelial cell adhesion. Nano Res, 9(11):3407–3427. https://doi.org/10.1007/s12274-016-1218-3
- Thompson JF, Soni BK, Weatherill NP, 1999, Handbook of Grid Generation, Boca Raton, FL, CRC Press. https://doi.org/10.1201/9781420050349
- Thakur RK, Vial C, Nigam KDP, et al., 2003, Static mixers in the process industries—A review, 81. https://doi.org/10.1205/026387603322302968
- Rafeie M, Welleweerd M, Hassanzadeh-Barforoushi A, et al., 2017, An easily fabricated three-dimensional threaded lemniscate-shaped micromixer for a wide range of flow rates. Biomicrofluidics, 11(1). https://doi.org/10.1063/1.4974904
- Santana HS, Silva JL, Taranto OP, 2015, Numerical simulation of mixing and reaction of Jatropha curcas oil and ethanol for synthesis of biodiesel in micromixers. Chem Eng Sci, 132:59–168. https://doi.org/10.1016/j.ces.2015.04.014
- Zhang J, Luo X, 2018, Mixing performance of a 3D micro T-mixer with swirl-inducing inlets and rectangular constriction. Micromachines, 9(5). https://doi.org/10.3390/mi9050199
- Shah I, Kim SW, Kim K, et al., 2019, Experimental and numerical analysis of Y-shaped split and recombination micro-mixer with different mixing units. Chem Eng J, 358:691–706. https://doi.org/10.1016/j.cej.2018.09.045
- Raza W, Hossain S, Kim KY, 2017, Effective mixing in a short serpentine split-and-recombination micromixer. Sensors Actuators B Chem, 258:381–392. https://dx.doi.org/10.1016/j.snb.2017.11.135
- Mouheb NA, Malsch D, Montillet A, et al., 2012, Numerical and experimental investigations of mixing in T-shaped and cross-shaped micromixers. Chem Eng Sci, 68(1):278–289. https://doi.org/10.1016/j.ces.2011.09.036
- Rauline D, Tanguy PA, Le Blévec JM, et al., 1998, Numerical investigation of the performance of several static mixers. The Canadian J Chem Eng, 76(3):527−535. https://doi.org/10.1002/cjce.5450760325
- Grace HP, 1982, Dispersion phenomena in high viscosity immiscible fluid systems and application of static mixers as dispersion devices in such systems. Chem Eng Commun, 14(3–6):225–277. https://doi.org/10.1080/00986448208911047
- Shah NF, Kale DD, 1991, Pressure drop for laminar flow of non-Newtonian fluids in static mixers. Chem Eng Sci, 46(8):2159–2161. https://doi.org/10.1016/0009-2509(91)80175-X
- Haddadi MM, Hosseini SH, Rashtchian D, et al., 2020, Comparative analysis of different static mixers performance by CFD technique: An innovative mixer. Chin J Chem Eng, 28(3):672–684. https://doi.org/10.1016/j.cjche.2019.09.004
- Ates G, Bartolo P, 2021, Numerical simulation of multimaterial polymer mixing for bioprinting applications. J Addit Manuf Technol, 1(3):606. https://10.18416/JAMTECH.2111606
- Hozumi T, Ohta S, Ito T, 2015, Analysis of the calcium alginate gelation process using a Kenics static mixer. Ind Eng Chem Res, 54(7):2099–2107. https://doi.org/10.1021/ie5044693
- Ortega ES, Sanz-Garcia A, Pernia-Espinoza A, et al., 2019, Efficient fabrication of polycaprolactone scaffolds for printing hybrid tissue-engineered constructs. Materials (Basel), 12(4):1–18. https://doi.org/10.3390/ma12040613
- Aguado BA, Mulyasasmita W, Su J, et al., 2012, Improving viability of stem cells during syringe needle flow through the design of hydrogel cell carriers. Tissue Eng Part A, 18(7-8): 806–815. https://doi.org/10.1089/ten.tea.2011.0391
- Gómez-Blanco JC, Mancha-Sánchez E, Marcos AC, et al., 2020, Bioink temperature influence on shear stress, pressure and velocity using computational simulation. Processes, 8(7):1–18. https://doi.org/10.3390/PR8070865
- Meng HB, Song MY, Yu YF, et al., 2017, Enhancement of laminar flow and mixing performance in a lightnin static mixer. Int J Chem React Eng, 15(3):1–21. https://doi.org/10.1515/ijcre-2016-0112
- Nair K, Gandhi M, Khalil S, et al., 2009, Characterization of cell viability during bioprinting processes. Biotechnol J, 4(8):1168–1177. https://doi.org/10.1002/biot.200900004
- Sarker M, Chen XB, 2017, Modeling the flow behavior and flow rate of medium viscosity alginate for scaffold fabrication with a three-dimensional bioplotter. J Manuf Sci Eng Trans ASME, 139(8):1–14. https://doi.org/10.1115/1.4036226
- Paxton N, Smolan W, Böck T, et al., 2017, Proposal to assess printability of bioinks for extrusion-based bioprinting and evaluation of rheological properties governing bioprintability. Biofabrication, 9(4). https://doi.org/10.1088/1758-5090/aa8dd8
- Snyder J, Son AR, Hamid Q, et al., 2015, Mesenchymal stem cell printing and process regulated cell properties. Biofabrication, 7(4). https://doi.org/10.1088/1758-5090/7/4/044106
- Liu W, Heinrich MA, Zhou Y, et al., 2017, Extrusion bioprinting of shear-thinning gelatin methacryloyl bioinks. Adv Healthc Mater, 6(12):1–11. https://doi.org/10.1002/adhm.201601451
- Blaeser A, Campos DFD, Puster U, et al., 2016, Controlling shear stress in 3D bioprinting is a key factor to balance printing resolution and stem cell integrity. Adv Healthc Mater, 5(3):326–333. https://doi.org/10.1002/adhm.201500677
- Manojlovic V, Djonlagic J, Obradovic B, et al., 2006, Investigations of cell immobilization in alginate: Rheological and electrostatic extrusion studies. J Chem Technol Biotechnol, 81(4):505–510. https://doi.org/10.1002/jctb.1465