A computational model of cell viability and proliferation of extrusion-based 3D-bioprinted constructs during tissue maturation process
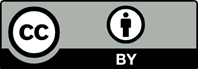
3D bioprinting is a novel promising solution for living tissue fabrication, with several potential advantages in many different applicative sectors. However, the implementation of complex vascular networks remains as one of the limiting factors for the production of complex tissues and for bioprinting scale-up. In this work, a physics-based computational model is presented to describe nutrients diffusion and consumption phenomena in bioprinted constructs. The model—a system of partial differential equations that is approximated by means of the finite element method— allows for the description of cell viability and proliferation, and it can be easily adapted to different cell types, densities, biomaterials, and 3D-printed geometries, thus allowing a preassessment of cell viability within the bioprinted construct. The experimental validation is performed on bioprinted specimens to assess the ability of the model to predict changes in cell viability. The proposed model constitutes a proof of concept of digital twinning of biofabricated constructs that can be suitably included in the basic toolkit for tissue bioprinting.
Ng WL, Chua CK., Shen YF, 2019, Print me an organ! Why we are not there yet. Progr Polym Sci, 97: 101–145. https://doi.org/10.1016/j.progpolymsci.2019.101145
Dey M, Ozbolat IT, 2020, 3D bioprinting of cells, tissues and organs. Sci Rep, 10(1): 14023. https://doi.org/10.1038/s41598-020-70086-y
Santoni S, Gugliandolo SG, Sponchioni M, et al., 2022, 3D bioprinting: Current status and trends—A guide to the literature and industrial practice. Bio-Des Manuf, 5(1): 14–42. https://doi.org/10.1007/s42242-021-00165-0
Alexander AE, Wake N, Chepelev L, et al., 2021, A guideline for 3D printing terminology in biomedical research utilizing ISO/ASTM standards. 3D Print Med, 7(1): 8. https://doi.org/10.1186/s41205-021-00098-5
Moroni L, Boland T, Burdock JA, et al., 2018, Biofabrication: A guide to technology and terminology. Trends Biotechnol, 36(4): 384–402. https://doi.org/10.1016/j.tibtech.2017.10.015
Ozbolat IT, Hospodiuk M, 2016, Current advances and future perspectives in extrusion-based bioprinting. Biomaterials, 76: 321–343. https://doi.org/10.1016/j.biomaterials.2015.10.076
Ng WL, Huang X, Shkolnikov V, et al., 2022, Controlling droplet impact velocity and droplet volume: Key factors to achieving high cell viability in sub-nanoliter droplet-based bioprinting. Int J Bioprint, 8(1): 1–17. https://doi.org/10.18063/IJB.V8I1.424
Long WL, Lee JM, Zhou M et al., 2020, Vat polymerization-based bioprinting—Process, materials, applications and regulatory challenges. Biofabrication, 12(2): 022001. https://doi.org/10.1088/1758-5090/ab6034
Piazza DE, Pandolfi E, Cacciotti I, et al., 2021, Bioprinting technology in skin, heart, pancreas and cartilage tissues: Progress and challenges in clinical practice. Int J Environ Res Public Health, 18(20): 10806. https://doi.org/10.3390/ijerph182010806
Zhu W, Qu X, Zhu J, et al. 2016, Analytic models of oxygen and nutrient diffusion, metabolism dynamics, and architecture optimization in three-dimensional tissue constructs with applications and insights in cerebral organoids. Tissue Eng Part C Methods, 22(3): 221–249. https://doi.org/10.1089/ten.TEC.2015.0375
Zhu W, Qu X, Zhu J, et al., 2017, Direct 3D bioprinting of prevascularized tissue constructs with complex microarchitecture. Biomaterials, 124: 106–115. https://doi.org/10.1016/j.biomaterials.2017.01.042
Ehsan SM, George SC, 2013, Nonsteady state oxygen transport in engineered tissue: Implications for design. Tissue Eng Part A, 19(11–12): 1433–1442. https://doi.org/10.1089/ten.tea.2012.0587
Magliaro C, Mattei G, Iacoangeli F, et al., 2019, Oxygen consumption characteristics in 3D constructs depend on cell density. Front Bioeng Biotechnol, 7: 251. https://doi.org/10.3389/fbioe.2019.00251
Jin H, Lei J, 2014, A mathematical model of cell population dynamics with autophagy response to starvation. Math Biosci, 258: 1–10. https://doi.org/10.1016/j.mbs.2014.08.014
Vogels M, Zoeckler R, Stasiw DM, et al., 1975, P.F. Verhulst’s ‘Notice sur la loi que la populations suit dans son accroissement’ from Correspondence Mathematique. Ghent, X:1838. J Biol Phys 3: 183–192. https://doi.org/10.1007/BF02309004
Ward JP, King JR, 1997, Mathematical modelling of avascular-tumour growth. [Online]. https://academic.oup.com/imammb/article/14/1/39/660000
Kiran KL, Jayachandran D, Lakshminarayanan S, 2009, Mathematical modelling of avascular tumour growth based on diffusion of nutrients and its validation. Can J Chem Eng, 87(5): 732–740. https://doi.org/10.1002/cjce.20204
Tindall MJ, Please CP, Peddie MJ, 2008, Modelling the formation of necrotic regions in avascular tumours. Math Biosci, 211(1): 34–55. https://doi.org/10.1016/j.mbs.2007.09.002
Fritz M, Lima EABF, Nikolić V, et al., 2019, Local and nonlocal phase-field models of tumor growth and invasion due to ECM degradation. Math Models Methods Appl Sci, 29(13): 2433–2468. https://doi.org/10.1142/S0218202519500519
Higuera G, Schop D, Janssen F, et al., Quantifying in vitro growth and metabolism kinetics of human mesenchymal stem cells using a mathematical model. [Online]. www. liebertpub.com
Xu P, 2020, Analytical solution for a hybrid Logistic-Monod cell growth model in batch and continuous stirred tank reactor culture. Biotechnol Bioeng, 117(3): 873–878. https://doi.org/10.1002/bit.27230
Quarteroni A, 2017, Numerical Models for Differential Problems, MS&A-Modeling, Simulation and Applications 16. [Online]. Accessed: December 06, 2022. https://link. springer.com/book/10.1007/978-3-319-49316-9
Petter Langtangen H, Logg A, 2017, Solving PDEs in Python—The FEniCS tutorial volume I.
Wagner BA, Venkataraman S, Buettner GR, 2011, The rate of oxygen utilization by cells. Free Radic Biol Med, 51(3): 700–712. https://doi.org/10.1016/j.freeradbiomed.2011.05.024
ThermoFisher Scientific. DMEM - Dulbecco’s Modified Eagle Medium. Available on-line. https://www.thermofisher.com/ order/catalog/product/11965092?SID=srch-srp-11965092.
Gupta SC, Gupta N, Ahlawat SPS, et al., 2005, In vitro culture of skin fibroblast cells for potential cloning by nuclear transfer, in Applications of Gene-Based Technologies for Improving Animal Production and Health in Developing Countries, Berlin/Heidelberg, Springer-Verlag, 631–640. https://doi.org/10.1007/1-4020-3312-5_47
Niu H, Li C, Guan Y, et al., 2020, High oxygen preservation hydrogels to augment cell survival under hypoxic condition. Acta Biomater, 105: 56–67. https://doi.org/10.1016/j.actbio.2020.01.017
Gruetter R, Ugurbil K, Seaquist ER, 1998, Steady-state cerebral glucose concentrations and transport in the human brain. J Neurochem, 70(1): 397–408. https://doi.org/10.1046/j.1471-4159.1998.70010397.x
Buchwald P, 2009, FEM-based oxygen consumption and cell viability models for avascular pancreatic islets. Theor Biol Med Model, 6(1): 5. https://doi.org/10.1186/1742-4682-6-5
CELLINK Series. [Online]. www.cellink.com
Dai LG, Dai NT, Chen TY, et al., 2022, A bioprinted vascularized skin substitute with fibroblasts, keratinocytes, and endothelial progenitor cells for skin wound healing. Bioprinting, 28: e00237 https://doi.org/10.1016/j.bprint.2022.e00237
Krishnamoorthy S, Zhang Z, Xu C, 2019, Biofabrication of three-dimensional cellular structures based on gelatin methacrylate–alginate interpenetrating network hydrogel. J Biomater Appl, 33(8): 1105–1117. https://doi.org/10.1177/0885328218823329
Yao B, Hu T, Cui X, et al., 2019, Enzymatically degradable alginate/gelatin bioink promotes cellular behavior and degradation in vitro and in vivo. Biofabrication, 11(4): 045020. https://doi.org/10.1088/1758-5090/ab38ef
Sarker B, Singh R, Zehnder T, et al., 2017, Macromolecular interactions in alginate-gelatin hydrogels regulate the behavior of human fibroblasts. J Bioact Compat Polym, 32(3): 309–324. https://doi.org/10.1177/0883911516668667
Zhang Y, Kumar P, LvS, et al., 2021, Recent advances in 3D bioprinting of vascularized tissues. Mater Des, 199: 109398. https://doi.org/10.1016/j.matdes.2020.109398
Novosel EC, Kleinhans C, Kluger PJ, 2011, Vascularization is the key challenge in tissue engineering. Adv Drug Deliv Rev, 63(4): 300–311. https://doi.org/10.1016/j.addr.2011.03.004
Li X, Liu L, Zhang X, et al., 2018, Research and development of 3D printed vasculature constructs. Biofabrication, 10(3). https://doi.org/10.1088/1758-5090/aabd56
Zhu J, Wang Y, Zhong L, et al., 2021, Advances in tissue engineering of vasculature through three-dimensional bioprinting. Dev Dynamics, 250(12): 1717–1738. https://doi.org/10.1002/dvdy.385