Applications of 3D printing in aging
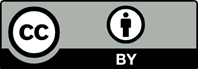
Aging is inevitable, and how to age healthily is a key concern. Additive manufacturing offers many solutions to this problem. In this paper, we first briefly introduce various 3D printing technologies commonly used in the biomedical field, particularly in aging research and aging care. Next, we closely examine aging-related health conditions of nervous system, musculoskeletal system, cardiovascular system, and digestive system with a focus on the application of 3D printing in these fields, including the creation of in vitro models and implants, production of drugs and drug delivery systems, and fabrication of rehabilitation and assistive medical devices. Finally, the opportunities, challenges, and prospects of 3D printing in the field of aging are discussed.
1. Giacomello E, Toniolo L, 2021, Nutrition, diet and healthy aging. Nutrients, 14(1):190. https://doi.org/10.3390/nu14010190
2. United Nations, 2022, World Population Prospects 2022, United Nations, Department of Economic and Social Affairs, Population Division.
3. Hou Y, Dan, X, Babbar M, et al., 2019, Ageing as a risk factor for neurodegenerative disease. Nat Rev Neurol, 15:565–581. https://doi.org/10.1038/s41582-019-0244-7
4. Guo J, Huang X, Dou L, et al., 2022, Aging and aging-related diseases: From molecular mechanisms to interventions and treatments. Signal Transduct Targ Ther, 7:391. https://doi.org/10.1038/s41392-022-01251-0
5. Niccoli T, Partridge L, 2012, Ageing as a risk factor for disease. Curr Biol, 22:R741–R752. https://doi.org/10.1016/j.cub.2012.07.024
6. Johnston CB, Dagar M, 2020, Osteoporosis in older adults. Med Clin North Am, 104:873–884. https://doi.org/10.1016/j.mcna.2020.06.004
7. Martel-Pelletier J, Barr A, Cicuttini F, et al., 2016, Osteoarthritis. Nat Rev Dis Primers, 2:16072. https://doi.org/10.1038/nrdp.2016.72
8. Avvedimento M, Tang GHL, 2021, Transcatheter aortic valve replacement (TAVR): Recent updates. Prog Cardiovasc Dis, 69:73–83. https://doi.org/10.1016/j.pcad.2021.11.003
9. Pinter J, Hanson Camilla S, Chapman JR, et al., 2017, Perspectives of older kidney transplant recipients on kidney transplantation. Clin J Am Soc Nephrol, 12:443–453. https://doi.org/10.2215/cjn.05890616
10. Zhu W, Ma X, Gou M, et al., 2016, 3D printing of functional biomaterials for tissue engineering. Curr Opin Biotechnol, 40:103–112. https://doi.org/10.1016/j.copbio.2016.03.014
11. Matai I, Kaur G, Seyedsalehi A, et al., 2020, Progress in 3D bioprinting technology for tissue/organ regenerative engineering. Biomaterials, 226:119536. https://doi.org/10.1016/j.biomaterials.2019.119536
12. Wang S, Zhao S, Yu J, et al., 2022, Advances in translational 3D printing for cartilage, bone, and osteochondral tissue engineering. Small, 18:e2201869. https://doi.org/10.1002/smll.202201869
13. Lozano R, Stevens L, Thompson BC, et al., 2015, 3D printing of layered brain-like structures using peptide modified gellan gum substrates. Biomaterials, 67:264–273. https://doi.org/10.1016/j.biomaterials.2015.07.022
14. Teng CL., Chen JY, Chang TL, et al., 2020, Design of photocurable, biodegradable scaffolds for liver lobule regeneration via digital light process-additive manufacturing. Biofabrication, 12:035024. https://doi.org/10.1088/1758-5090/ab69da
15. Yanagi Y, Nakayama K, Taguchi T, et al., 2017, In vivo and ex vivo methods of growing a liver bud through tissue connection. Sci Rep, 7:14085. https://doi.org/10.1038/s41598-017-14542-2
16. Zhou X, Tenaglio S, Esworthy T, et al., 2020, Three-dimensional printing biologically inspired DNA-based gradient scaffolds for cartilage tissue regeneration. ACS Appl Mater Interfaces, 12:33219–33228. https://doi.org/10.1021/acsami.0c07918
17. Li P, Fu L, Liao Z, et al., 2021, Chitosan hydrogel/3D-printed poly(ε-caprolactone) hybrid scaffold containing synovial mesenchymal stem cells for cartilage regeneration based on tetrahedral framework nucleic acid recruitment. Biomaterials, 278:121131. https://doi.org/10.1016/j.biomaterials.2021.121131
18. Ma C, Li W, Li D, et al., 2022, Photoacoustic imaging of 3D-printed vascular networks. Biofabrication, 14:025001. https://doi.org/10.1088/1758-5090/ac49d5
19. Jia W, Gungor-Ozkerim PS, Zhang YS, et al., 2016, Direct 3D bioprinting of perfusable vascular constructs using a blend bioink. Biomaterials, 106:58–68. https://doi.org/10.1016/j.biomaterials.2016.07.038
20. Krieger KJ, Bertollo N, Dangol M, et al., 2019, Simple and customizable method for fabrication of high-aspect ratio microneedle molds using low-cost 3D printing. Microsyst Nanoeng, 5:42. https://doi.org/10.1038/s41378-019-0088-8
21. Choo S, Jin S, Jung J, 2022, Fabricating high-resolution and high-dimensional microneedle mold through the resolution improvement of stereolithography 3D printing. Pharmaceutics, 14(4):766. https://doi.org/10.3390/pharmaceutics14040766
22. Lorenz T, Iskandar MM, Baeghbali V, et al., 2022, 3D food printing applications related to dysphagia: A narrative review. Foods, 11(12):1789. https://doi.org/10.3390/foods11121789
23. Giura L, Urtasun L, Belarra A, et al., 2021, Exploring tools for designing dysphagia-friendly foods: A review. Foods, 10(6):1334. https://doi.org/10.3390/foods10061334
24. Ligon SC, Liska R, Stampfl J, et al., 2017, Polymers for 3D printing and customized additive manufacturing. Chem Rev, 117:10212–10290. https://doi.org/10.1021/acs.chemrev.7b00074
25. Cailleaux S, Sanchez-Ballester NM, Gueche YA, et al., 2021, Fused deposition modeling (FDM), the new asset for the production of tailored medicines. J Control Release, 330:821–841. https://doi.org/10.1016/j.jconrel.2020.10.056
26. Frölich AM, Spallek J, Brehmer L et al., 2016, 3D printing of intracranial aneurysms using fused deposition modeling offers highly accurate replications. AJNR Am J Neuroradiol, 37:120–124. https://doi.org/10.3174/ajnr.A4486
27. Mowry SE, Jammal H, Myer Ct, et al., 2015, A novel temporal bone simulation model using 3D printing techniques. Otol Neurotol, 36:1562–1565. https://doi.org/10.1097/mao.0000000000000848
28. Pucci JU, Christophe BR, Sisti JA, et al., 2017, Three-dimensional printing: Technologies, applications, and limitations in neurosurgery. Biotechnol Adv, 35:521–529. https://doi.org/10.1016/j.biotechadv.2017.05.007
29. Goyanes A, Allahham N, Trenfield SJ, et al., 2019, Direct powder extrusion 3D printing: Fabrication of drug products using a novel single-step process. Int J Pharmaceut, 567:118471. https://doi.org/10.1016/j.ijpharm.2019.118471
30. La Gala A, Fiorio R, Ceretti DVA, et al., 2021, A combined experimental and modeling study for pellet-fed extrusion-based additive manufacturing to evaluate the impact of the melting efficiency. Materials, 14(19):5566. https://doi.org/10.3390/ma14195566
31. Xiong J, Wang H, Lan X, et al., 2022, Fabrication of bioinspired grid-crimp micropatterns by melt electrospinning writing for bone-ligament interface study. Biofabrication, 14:025008. https://doi.org/10.1088/1758-5090/ac4ac8
32. Su Y, Qiu T, Song W, et al., 2021, Melt electrospinning writing of magnetic microrobots. Adv Sci, 8:2003177. https://doi.org/10.1002/advs.202003177
33. Schipani R, Scheurer S, Florentin R, et al., 2020, Reinforcing interpenetrating network hydrogels with 3D printed polymer networks to engineer cartilage mimetic composites. Biofabrication, 12:035011. https://doi.org/10.1088/1758-5090/ab8708
34. Coburn J, Gibson M, Bandalini PA, et al., 2011, Biomimetics of the extracellular matrix: An integrated three-dimensional fiber-hydrogel composite for cartilage tissue engineering. Smart Struct Syst, 7:213–222. https://doi.org/10.12989/sss.2011.7.3.213
35. Lewis JA, 2006, Direct ink writing of 3D functional materials. Adv Funct Mater, 16:2193–2204. https://doi.org/10.1002/adfm.200600434
36. Qian F, Zhu C, Knipe JM, et al., 2019, Direct writing of tunable living inks for bioprocess intensification. Nano Lett, 19:5829–5835. https://doi.org/10.1021/acs.nanolett.9b00066
37. Grottkau BE, Hui ZX, Pang YG, 2020, A novel 3D bioprinter using direct-volumetric drop-on-demand technology for fabricating micro-tissues and drug-delivery. Int J Mol Sci, 21(10):3482. https://doi.org/10.3390/ijms21103482
38. Cameron T, Naseri E, MacCallum B, et al., 2020, Development of a disposable single-nozzle printhead for 3D bioprinting of continuous multi-material constructs. Micromachines, 11(5):459. https://doi.org/10.3390/mi11050459
39. Seiti M, Ginestra P, Ferraro RM, et al., 2020, Nebulized jet-based printing of bio-electrical scaffolds for neural tissue engineering: A feasibility study. Biofabrication, 12:025024. https://doi.org/10.1088/1758-5090/ab71e0
40. Suntornnond R, Ng WL, Huang X, et al., 2022, Improving printability of hydrogel-based bio-inks for thermal inkjet bioprinting applications via saponification and heat treatment processes. J Mater Chem B, 10:5989–6000. https://doi.org/10.1039/d2tb00442a
41. Cui X, Dean D, Ruggeri ZM, et al., 2010, Cell damage evaluation of thermal inkjet printed Chinese hamster ovary cells. Biotechnol Bioeng, 106:963–969. https://doi.org/10.1002/bit.22762
42. Popov VK, Evseev AV, Ivanov AL, et al., 2004, Laser stereolithography and supercritical fluid processing for custom-designed implant fabrication. J Mater Sci Mater Med, 15:123–128. https://doi.org/10.1023/b:jmsm.0000011812.08185.2a
43. Xu X, Awad A, Robles-Martinez P, et al., 2021, Vat photopolymerization 3D printing for advanced drug delivery and medical device applications. J Controll Release, 329:743–757. https://doi.org/10.1016/j.jconrel.2020.10.008
44. Kim SH, Hong H, Ajiteru O, et al., 2021, 3D bioprinted silk fibroin hydrogels for tissue engineering. Nat Protoc, 16:5484–5532. https://doi.org/10.1038/s41596-021-00622-1
45. Song JX, Michas C, Chen CS, et al., 2020, From simple to architecturally complex hydrogel scaffolds for cell and tissue engineering applications: Opportunities presented by two-photon polymerization. Adv Healthc Mater, 9:1901217. https://doi.org/10.1002/adhm.201901217
46. Carlotti M, Mattoli V, 2019, Functional materials for two-photon polymerization in microfabrication. Small, 15:1902687. https://doi.org/10.1002/smll.201902687
47. Torgersen J, Qin X-H, Li Z, et al., 2013, Hydrogels for two-photon polymerization: A toolbox for mimicking the extracellular matrix. Adv Funct Mater, 23:4542–4554. https://doi.org/10.1002/adfm.201203880
48. Ciuciu AI, Cywinski PJ, 2014, Two-photon polymerization of hydrogels—Versatile solutions to fabricate well-defined 3D structures. Rsc Adv, 4:45504–45516. https://doi.org/10.1039/c4ra06892k
49. Mussatto A, Groarke R, O’Neill A, et al., 2021, Influences of powder morphology and spreading parameters on the powder bed topography uniformity in powder bed fusion metal additive manufacturing. Addit Manuf, 38:101807. https://doi.org/10.1016/j.addma.2020.101807
50. Awad A, Fina F, Goyanes A, et al., 2021, Advances in powder bed fusion 3D printing in drug delivery and healthcare. Adv Drug Deliv Rev, 174:406–424. https://doi.org/10.1016/j.addr.2021.04.025
51. Chen W, Yang J, Kong H, et al., 2021, Fatigue behaviour and biocompatibility of additively manufactured bioactive tantalum graded lattice structures for load-bearing orthopaedic applications. Mater Sci Eng C Mater Biol Appl, 130:112461. https://doi.org/10.1016/j.msec.2021.112461
52. Zhao D, Liang H, Han C, et al., 2021, 3D printing of a titanium-tantalum gyroid scaffold with superb elastic admissible strain, bioactivity and in-situ bone regeneration capability. Addit Manuf, 47:102223. https://doi.org/10.1016/j.addma.2021.102223
53. Asefy Z, Hoseinnejhad S, Ceferov Z, 2021, Nanoparticles approaches in neurodegenerative diseases diagnosis and treatment. Neurol Sci, 42:2653–2660. https://doi.org/10.1007/s10072-021-05234-x
54. Alzheimer’s A, et al., 2019, 2019 Alzheimer’s disease facts and figures. Alzheimers Dement, 15:321–387. https://doi.org/10.1016/j.jalz.2019.01.010
55. Poewe W, Seppi K, Tanner C, et al., 2017, Parkinson disease. Nat Rev Dis Prim, 3:17013 . https://doi.org/10.1038/nrdp.2017.13
56. Nussbaum RL, Ellis CE, 2003, Genomic medicine: Alzheimer’s disease and Parkinson’s disease. N Engl J Med, 348:1356–1364. https://doi.org/10.1056/NEJM2003ra020003
57. Mehta P, Antao V, Sanchez M, et al., 2014, Prevalence of amyotrophic lateral sclerosis (ALS) in the United States. Ann Neurol, 76, S64–S64.
58. Hsiao YH, Chang CH, Gean PW, 2018, Impact of social relationships on Alzheimer’s memory impairment: Mechanistic studies. J Biomed Sci, 25:3. https://doi.org/10.1186/s12929-018-0404-x
59. Ross CA, Poirier MA, 2004, Protein aggregation and neurodegenerative disease. Nat Med, 10(Suppl):S10–S17. https://doi.org/10.1038/nm1066
60. Mattson MP, Cheng B, Davis D, et al., 2019, Apolipoprotein E and Alzheimer disease: Pathobiology and targeting strategies. Nat Rev Neurol, 15:501–518. https://doi.org/10.1038/s41582-019-0228-7
61. Mattson MP, et al., 1992, beta-Amyloid peptides destabilize calcium homeostasis and render human cortical neurons vulnerable to excitotoxicity. J Neurosci, 12:376–389. https://doi.org/10.1523/jneurosci.12-02-00376.1992
62. Citron M, Westaway D, Xia W, et al., 1997, Mutant presenilins of Alzheimer’s disease increase production of 42-residue amyloid beta-protein in both transfected cells and transgenic mice. Nat Med, 3:67–72. https://doi.org/10.1038/nm0197-67
63. Bloom GS, 2014, Amyloid-β and tau: The trigger and bullet in Alzheimer disease pathogenesis. JAMA Neurol, 71:505–508. https://doi.org/10.1001/jamaneurol.2013.5847
64. Selkoe DJ, 2001, Alzheimer’s disease: Genes, proteins, and therapy. Physiol Rev, 81:741–766. https://doi.org/10.1152/physrev.2001.81.2.741
65. Tolosa E, Garrido A, Scholz SW, et al., 2021, Challenges in the diagnosis of Parkinson’s disease. Lancet Neurol, 20:385–397. https://doi.org/10.1016/s1474-4422(21)00030-2
66. Choudhury SP, Bano S, Sen S, et al., 2022, Altered neural cell junctions and ion-channels leading to disrupted neuron communication in Parkinson’s disease. NPJ Parkinsons Dis, 8:66. https://doi.org/10.1038/s41531-022-00324-9
67. de Lau LM, Breteler MM, 2006, Epidemiology of Parkinson’s disease. Lancet Neurol, 5:525–535. https://doi.org/10.1016/s1474-4422(06)70471-9
68. Al-Chalabi A, Hardiman O, 2013, The epidemiology of ALS: A conspiracy of genes, environment and time. Nat Rev Neurol, 9:617–628. https://doi.org/10.1038/nrneurol.2013.203
69. Yun Y, Ha Y, 2020, CRISPR/Cas9-mediated gene correction to understand ALS. Int J Mol Sci, 21. https://doi.org/10.3390/ijms21113801
70. Yang X, Ji Y, Wang W, et al., Amyotrophic lateral sclerosis: Molecular mechanisms, biomarkers, and therapeutic strategies. Antioxidants (Basel), 10(7):1012. https://doi.org/10.3390/antiox10071012
71. Selim OA, Lakhani S, Midha S, et al., 2022, Three-dimensional engineered peripheral nerve: Toward a new era of patient-specific nerve repair solutions. Tissue Eng Part B Rev, 28:295–335. https://doi.org/10.1089/ten.TEB.2020.0355
72. Zhuang P, Sun AX, An J, et al., 2018, 3D neural tissue models: From spheroids to bioprinting. Biomaterials, 154:113–133. https://doi.org/10.1016/j.biomaterials.2017.10.002
73. Shamir ER, Ewald AJ, 2014, Three-dimensional organotypic culture: experimental models of mammalian biology and disease. Nat Rev Mol Cell Biol, 15:647–664. https://doi.org/10.1038/nrm3873
74. Zhang D, Pekkanen-Mattila M, Shahsavani M, et al., 2014, A 3D Alzheimer’s disease culture model and the induction of P21-activated kinase mediated sensing in iPSC derived neurons. Biomaterials, 35:1420–1428. https://doi.org/10.1016/j.biomaterials.2013.11.028
75. Kelava I, Lancaster MA, 2016, Stem cell models of human brain development. Cell Stem Cell, 18:736–748. https://doi.org/10.1016/j.stem.2016.05.022
76. Kato-Negishi M, Morimoto Y, Onoe H, et al., 2013, Millimeter-sized neural building blocks for 3D heterogeneous neural network assembly. Adv Healthc Mater, 2:1564–1570. https://doi.org/10.1002/adhm.201300052
77. Jo J, Xiao Y, Sun AX, et al., 2016, Midbrain-like organoids from human pluripotent stem cells contain functional dopaminergic and neuromelanin-producing neurons. Cell Stem Cell, 19:248–257. https://doi.org/10.1016/j.stem.2016.07.005
78. Marton RM, Pașca SP, 2020, Organoid and assembloid technologies for investigating cellular crosstalk in human brain development and disease. Trends Cell Biol, 30:133–143. https://doi.org/10.1016/j.tcb.2019.11.004
79. Gu Q, Tomaskovic-Crook E, Lozano R, et al., 2016, Functional 3D neural mini-tissues from printed gel-based bioink and human neural stem cells. Adv Healthc Mater, 5:1429–1438. https://doi.org/10.1002/adhm.201600095
80. Zhou X, Cui H, Nowicki M, et al., 2018, Three-dimensional-bioprinted dopamine-based matrix for promoting neural regeneration. ACS Appl Mater Interfaces, 10:8993–9001. https://doi.org/10.1021/acsami.7b18197
81. Dong M, Wang X, Chen XZ, et al., 2020, 3D-printed soft magnetoelectric microswimmers for delivery and differentiation of neuron-like cells. Adv Funct Mater, 30:1910323. https://doi.org/10.1002/adfm.201910323
82. Gultekin HE, Tort S, Acartuerk F, 2022, Fabrication of three dimensional printed tablets in flexible doses: A comprehensive study from design to evaluation. J Drug Deliv Sci Technol, 74:103538. https://doi.org/10.1016/j.jddst.2022.103538
83. Saylam E, Akkaya Y, Ilhan E, et al., 2021, Levodopa-loaded 3D-printed poly (lactic) acid/chitosan neural tissue scaffold as a promising drug delivery system for the treatment of Parkinson’s disease. Appl Sci Basel, 11(22):10727. https://doi.org/10.3390/app112210727
84. Renishaw, 2020, Renishaw announces completion of clinical trial extension study, using its innovative drug delivery system in the investigation of CDNF as a treatment for Parkinson’s disease. https://www.renishaw.com.cn/zh/renishaw-announces-completion-of-clinical-trial-extension-study-using-its-innovative-drug-delivery-system-in-the-investigation-of-cdnf-as-a-treatment-for-parkinsons-disease--45590
85. Zhou Y, Jenkins ME, Naish MD, et al., 2018, in Development of a Wearable Tremor Suppression Glove. in 7th IEEE International Conference on Biomedical Robotics and Biomechatronics (BIOROB), 640–645.
86. Farshid SP, Eric W, Shwetak P, et al., 2019, AuraRing: Precise Electromagnetic Finger Tracking. in 32nd Annual ACM Symposium on User Interface Software and Technology (UIST), 122–124.
87. Wu YY, Acharya D, Xu C, et al., 2018, Custom-fit three-dimensional-printed BiPAP mask to improve compliance in patients requiring long-term noninvasive ventilatory support. J Med Devices Trans ASME, 12(3):031003. https://doi.org/10.1115/1.4040187
88. LeBoff MS, Greenspan SL, Insogna KL, et al., 2022, The clinician’s guide to prevention and treatment of osteoporosis. Osteoporos Int, 33:2049–2102. https://doi.org/10.1007/s00198-021-05900-y
89. Mundy GR, 2007, Osteoporosis and inflammation. Nutr Rev, 65:S147–S151. https://doi.org/10.1111/j.1753-4887.2007.tb00353.x
90. Reid IR, 2020, A broader strategy for osteoporosis interventions. Nat Rev Endocrinol, 16:333–339. https://doi.org/10.1038/s41574-020-0339-7
91. Słupski W, Jawień P, Nowak B, 2021, Botanicals in postmenopausal osteoporosis. Nutrients, 13(5):1609. https://doi.org/10.3390/nu13051609
92. Kendler DL, Marin F, Zerbini CAF, et al., 2018, Effects of teriparatide and risedronate on new fractures in post-menopausal women with severe osteoporosis (VERO): A multicentre, double-blind, double-dummy, randomised controlled trial. Lancet, 391:230–240. https://doi.org/10.1016/s0140-6736(17)32137-2
93. Burr DB, Gallant MA, 2012, Bone remodelling in osteoarthritis. Nat Rev Rheumatol, 8:665–673. https://doi.org/10.1038/nrrheum.2012.130
94. Felson DT, Lawrence RC, Dieppe PA, et al., 2000, Osteoarthritis: New insights. Part 1: The disease and its risk factors. Ann Intern Med, 133:635–646. https://doi.org/10.7326/0003-4819-133-8-200010170-00016
95. Cross M, Smith E, Hoy D, et al., 2014, The global burden of hip and knee osteoarthritis: estimates from the global burden of disease 2010 study. Ann Rheum Dis, 73: 1323–1330. https://doi.org/10.1136/annrheumdis-2013-204763
96. Hiligsmann M, Cooper C, Arden N, et al., 2013, Health economics in the field of osteoarthritis: An expert’s consensus paper from the European Society for Clinical and Economic Aspects of Osteoporosis and Osteoarthritis (ESCEO). Semin Arthritis Rheum, 43:303–313. https://doi.org/10.1016/j.semarthrit.2013.07.003
97. Loughlin J, 2022, Translating osteoarthritis genetics research: challenging times ahead. Trends Mol Med, 28:176–182. https://doi.org/10.1016/j.molmed.2021.12.007
98. Huang Z, He Z, Kong Y, et al., 2020, Insight into osteoarthritis through integrative analysis of metabolomics and transcriptomics. Clin Chim Acta, 510:323–329. https://doi.org/10.1016/j.cca.2020.07.010
99. Jiang W, Liu H, Wan R, et al., 2021, Mechanisms linking mitochondrial mechanotransduction and chondrocyte biology in the pathogenesis of osteoarthritis. Ageing Res Rev, 67:101315. https://doi.org/10.1016/j.arr.2021.101315
100. Zhou Q, Cai Y, Jiang Y, et al., 2020, Exosomes in osteoarthritis and cartilage injury: Advanced development and potential therapeutic strategies. Int J Biol Sci, 16:1811–1820. https://doi.org/10.7150/ijbs.41637
101. Katz JN, Arant KR, Loeser RF, 2021, Diagnosis and treatment of hip and knee osteoarthritis: A review. JAMA, 325:568–578. https://doi.org/10.1001/jama.2020.22171
102. Marshall M, Watt FE, Vincent TL, et al., 2018, Hand osteoarthritis: Clinical phenotypes, molecular mechanisms and disease management. Nat Rev Rheumatol, 14:641–656. https://doi.org/10.1038/s41584-018-0095-4
103. O’Neill TW, McCabe PS, McBeth J, 2018, Update on the epidemiology, risk factors and disease outcomes of osteoarthritis. Best Pract Res Clin Rheumatol, 32:312–326. https://doi.org/10.1016/j.berh.2018.10.007
104. Ma L, Wang X, Zhou Y, et al., 2021, Biomimetic Ti-6Al-4V alloy/gelatin methacrylate hybrid scaffold with enhanced osteogenic and angiogenic capabilities for large bone defect restoration. Bioact Mater, 6:3437–3448. https://doi.org/10.1016/j.bioactmat.2021.03.010
105. Wu N, Liu J, Ma W, et al., 2021, Degradable calcium deficient hydroxyapatite/poly(lactic-glycolic acid copolymer) bilayer scaffold through integral molding 3D printing for bone defect repair. Biofabrication, 13:025005. https://doi.org/10.1088/1758-5090/abcb48
106. Van HD Liang B, Anania S, et al., 2022, 3D-printed synthetic hydroxyapatite scaffold with in silico optimized macrostructure enhances bone formation in vivo. Adv Funct Mater, 32. https://doi.org/10.1002/adfm.202105002
107. Guillaume O, Geven MA, Sprecher CM, et al., 2017, Surface-enrichment with hydroxyapatite nanoparticles in stereolithography-fabricated composite polymer scaffolds promotes bone repair. Acta Biomater, 54:386–398. https://doi.org/10.1016/j.actbio.2017.03.006
108. Zhang B, Gui X, Song P, et al., 2022, Three-dimensional printing of large-scale, high-resolution bioceramics with micronano inner porosity and customized surface characterization design for bone regeneration. ACS Appl Mater Interfaces, 14:8804–8815. https://doi.org/10.1021/acsami.1c22868
109. Ye X, Li L, Lin Z, et al., 2018, Integrating 3D-printed PHBV/ calcium sulfate hemihydrate scaffold and chitosan hydrogel for enhanced osteogenic property. Carbohydr Polym, 202:106–114. https://doi.org/10.1016/j.carbpol.2018.08.117
110. Ratheesh G, Shi MC, Lau P, et al., 2021, Effect of dual pore size architecture on in vitro osteogenic differentiation in additively manufactured hierarchical scaffolds. ACS Biomater Sci Eng, 7:2615–2626. https://doi.org/10.1021/acsbiomaterials.0c01719
111. Wang W, Xiong Y, Zhao R, et al., 2022, A novel hierarchical biofunctionalized 3D-printed porous Ti6Al4V scaffold with enhanced osteoporotic osseointegration through osteoimmunomodulation. J Nanobiotechnol, 20:68. https://doi.org/10.1186/s12951-022-01277-0
112. Wang X, Li Z, Wang Z, et al., 2021, Incorporation of bone morphogenetic protein-2 and osteoprotegerin in 3D-printed Ti6Al4V scaffolds enhances osseointegration under osteoporotic conditions. Front Bioeng Biotechnol, 9:754205. https://doi.org/10.3389/fbioe.2021.754205
113. Cui Y, Wang Z, Li Z, et al., 2021, Functionalized anti-osteoporosis drug delivery system enhances osseointegration of an inorganic-organic bioactive interface in osteoporotic microenvironment. Mater Des, 206:109753. https://doi.org/10.1016/j.matdes.2021.109753
114. Li Z, Bai H, Wang Z, et al., 2022, Ultrasound-mediated rapamycin delivery for promoting osseointegration of 3D printed prosthetic interfaces via autophagy regulation in osteoporosis. Mater Des, 216:110586. https://doi.org/10.1016/j.matdes.2022.110586
115. Benders KE, van Weeren PR, Badylak SF, et al., 2013, Extracellular matrix scaffolds for cartilage and bone regeneration. Trends Biotechnol, 31:169–176. https://doi.org/10.1016/j.tibtech.2012.12.004
116. You F, Wu X, Zhu N, et al., 2016, 3D Printing of porous cell-laden hydrogel constructs for potential applications in cartilage tissue engineering. ACS Biomater Sci Eng, 2:1200– 1210. https://doi.org/10.1021/acsbiomaterials.6b00258
117. Rathan S, Dejob L, Schipani R, et al., 2019, Fiber reinforced cartilage ECM functionalized bioinks for functional cartilage tissue engineering. Adv Healthc Mater, 8, e1801501. https://doi.org/10.1002/adhm.201801501
118. Guan J, Yuan FZ, Mao ZM, et al., 2021, Fabrication of 3D-printed interpenetrating hydrogel scaffolds for promoting chondrogenic differentiation. Polymers (Basel), 13(13):2146. https://doi.org/10.3390/polym13132146
119. Visser J, Melchels FP, Jeon JE, et al., 2015, Reinforcement of hydrogels using three-dimensionally printed microfibres. Nat Commun, 6:6933. https://doi.org/10.1038/ncomms7933
120. Weiming C, Yong X, Yaqiang L, et al., 2020, 3D printing electrospinning fiber-reinforced decellularized extracellular matrix for cartilage regeneration. Chem Eng J, 382:122986. https://doi.org/10.1016/j.cej.2019.122986
121. Bas O, De-Juan-Pardo EM, et al., 2017, Biofabricated soft network composites for cartilage tissue engineering. Biofabrication, 9(2):025014. https://doi.org/10.1088/1758-5090/aa6b15
122. Mozaffarian D, Benjamin EJ, Go AS, et al., 2016, Heart disease and stroke statistics-2016 update: A report from the American Heart Association. Circulation, 133, e38–e360. https://doi.org/10.1161/cir.0000000000000350
123. Go AS, Mozaffarian D, Roger VL, et al., 2014, Heart disease and stroke statistics--2014 update: A report from the American Heart Association. Circulation, 129, e28–e292. https://doi.org/10.1161/01.cir.0000441139.02102.80
124. Leong DP, Joseph PG, McKee M, et al., 2017, Reducing the global burden of cardiovascular disease, Part 2: Prevention and treatment of cardiovascular disease. Circ Res, 121:695–710. https://doi.org/10.1161/circresaha.117.311849
125. Rumsfeld JS, Alexander KP, Goff DC, et al., 2013, Cardiovascular health: The importance of measuring patient-reported health status: A scientific statement from the American Heart Association. Circulation, 127:2233–2249. https://doi.org/10.1161/CIR.0b013e3182949a2e
126. Oliveira GMM, Brant LCC, Polanczyk CA, et al., 2022, Cardiovascular Statistics—Brazil 2021. Arq Brasil Cardiol, 118:115–372. https://doi.org/10.36660/abc.20211012
127. Liberale L, Montecucco F, Tardif JC, et al., 2020, Inflamm-ageing: The role of inflammation in age-dependent cardiovascular disease. Eur Heart J, 41: 2974–2982. https://doi.org/10.1093/eurheartj/ehz961
128. Jiang F, Yin K, Wu K, et al., 2021, The mechanosensitive Piezo1 channel mediates heart mechano-chemo transduction. Nat Commun, 12:869. https://doi.org/10.1038/s41467-021-21178-4
129. Ritchie RH, Abel ED, 2020, Basic mechanisms of diabetic heart disease. Circ Res, 126:1501–1525. https://doi.org/10.1161/circresaha.120.315913
130. Shirakabe A, Ikeda Y, Sciarretta S, et al., 2016, Aging and autophagy in the heart. Circ Res, 118:1563–1576. https://doi.org/10.1161/circresaha.116.307474
131. Ren J, Wu NN, Wang S, et al., 2021, Obesity cardiomyopathy: Evidence, mechanisms, and therapeutic implications. Physiol Rev, 101:1745–1807. https://doi.org/10.1152/physrev.00030.2020
132. Sun Z, 2015, Aging, arterial stiffness, and hypertension. Hypertension, 65:252–256. https://doi.org/10.1161/hypertensionaha.114.03617
133. Tracy EP, Hughes W, Beare JE, et al., 2021, Aging-induced impairment of vascular function: Mitochondrial redox contributions and physiological/clinical implications. Antioxid Redox Signal, 35:974–1015. https://doi.org/10.1089/ars.2021.0031
134. Donato AJ, Machin DR, Lesniewski LA, 2018, Mechanisms of dysfunction in the aging vasculature and role in age-related disease. Circ Res, 123:825–848. https://doi.org/10.1161/circresaha.118.312563
135. Ungvari Z, Tarantini S, Donato AJ, et al., 2018, Mechanisms of vascular aging. Circ Res, 123:849–867. https://doi.org/10.1161/circresaha.118.311378
136. Harvey A, Montezano AC, Lopes RA, et al., 2016, Vascular fibrosis in aging and hypertension: Molecular mechanisms and clinical implications. Can J Cardiol, 32: 659–668. https://doi.org/10.1016/j.cjca.2016.02.070
137. Jia G, Aroor AR, Jia C, et al., 2019, Endothelial cell senescence in aging-related vascular dysfunction. Biochim Biophys Acta Mol Basis Dis, 1865:1802–1809. https://doi.org/10.1016/j.bbadis.2018.08.008
138. El Assar M, Angulo J, Rodríguez-Mañas L, 2013, Oxidative stress and vascular inflammation in aging. Free Radic Biol Med, 65:380–401. https://doi.org/10.1016/j.freeradbiomed.2013.07.003
139. Alonzo M, AnilKumar S, Roman B, et al., 2019, 3D Bioprinting of cardiac tissue and cardiac stem cell therapy. Transl Res, 211:64–83. https://doi.org/10.1016/j.trsl.2019.04.004
140. Cui H, Miao S, Esworthy T, et al., 2018, 3D bioprinting for cardiovascular regeneration and pharmacology. Adv Drug Deliv Rev, 132:252–269. https://doi.org/10.1016/j.addr.2018.07.014
141. Gaudino M, Benedetto U, Fremes S, et al., 2018, Radial-artery or saphenous-vein grafts in coronary-artery bypass surgery. N Engl J Med, 378:2069–2077. https://doi.org/10.1056/NEJMoa1716026
142. Garekar S, Bharati A, Chokhandre M, et al., 2016, Clinical application and multidisciplinary assessment of three dimensional printing in double outlet right ventricle with remote ventricular septal defect. World J Pediatr Congenit Heart Surg, 7:344–350. https://doi.org/10.1177/2150135116645604
143. Maragiannis D, Jackson MS, Igo SR, et al., 2015, Replicating patient-specific severe aortic valve stenosis with functional 3D modeling. Circ Cardiovasc Imaging, 8:e003626. https://doi.org/10.1161/circimaging.115.003626
144. Jang J, Park HJ, Kim SW, et al., 2017, 3D printed complex tissue construct using stem cell-laden decellularized extracellular matrix bioinks for cardiac repair. Biomaterials, 112:264–274. https://doi.org/10.1016/j.biomaterials.2016.10.026
145. Noor N, Shapira A, Edri R, et al., 2019, 3D printing of personalized thick and perfusable cardiac patches and hearts. Adv Sci, 6(11):1900344. https://doi.org/10.1002/advs.201900344
146. Wu X, Chen K, Chai Q, et al., 2022, Freestanding vascular scaffolds engineered by direct 3D printing with Gt-Alg- MMT bioinks. Biomater Adv, 133:112658. https://doi.org/10.1016/j.msec.2022.112658
147. Sang JL, Ha HJ, Kyung SL, et al., 2019, Heparin coating on 3D printed poly (l-lactic acid) biodegradable cardiovascular stent via mild surface modification approach for coronary artery implantation. Chem Eng J, 378:122116. https://doi.org/10.1016/j.cej.2019.122116
148. Alonzo M, El Khoury R, Nagiah N, et al., 2022, 3D biofabrication of a cardiac tissue construct for sustained longevity and function. ACS Appl Mater Interfaces, 14:21800–21813. https://doi.org/10.1021/acsami.1c23883
149. Lee A, Hudson AR, Shiwarski DJ, et al., 2019, 3D bioprinting of collagen to rebuild components of the human heart. Science, 365:482–487. https://doi.org/10.1126/science.aav9051
150. Marcenes W, Kassebaum NJ, Bernabé E, et al., 2013, Global burden of oral conditions in 1990-2010: A systematic analysis. J Dent Res, 92:592–597. https://doi.org/10.1177/0022034513490168
151. Michaud DS, Fu Z, Shi J, et al., 2017, Periodontal disease, tooth loss, and cancer risk. Epidemiol Rev, 39:49–58. https://doi.org/10.1093/epirev/mxx006
152. Tanaka T, Takahashi K, Hirano H, et al., 2018, Oral frailty as a risk factor for physical frailty and mortality in community-dwelling elderly. J Gerontol A Biol Sci Med Sci, 73:1661–1667. https://doi.org/10.1093/gerona/glx225
153. Könönen E, Gursoy M, Gursoy UK, 2019, Periodontitis: A multifaceted disease of tooth-supporting tissues. J Clin Med, 8(8):1135. https://doi.org/10.3390/jcm8081135
154. Frencken JE, Sharma P, Stenhouse L, et al., 2017, Global epidemiology of dental caries and severe periodontitis—A comprehensive review. J Clin Periodontol, 44(Suppl 18): S94–S105. https://doi.org/10.1111/jcpe.12677
155. Stansbury JW, Idacavage MJ, 2016, 3D printing with polymers: Challenges among expanding options and opportunities. Dental Mater, 32:54–64. https://doi.org/10.1016/j.dental.2015.09.018
156. Della Bona A, Cantelli V, Britto VT, et al., 2021, 3D printing restorative materials using a stereolithographic technique: A systematic review. Dent Mater, 37:336–350. https://doi.org/10.1016/j.dental.2020.11.030
157. Amrollahi P, Shah B, Seifi A, et al., 2016, Recent advancements in regenerative dentistry: A review. Mater Sci Eng C Mater Biol Appl, 69:1383–1390. https://doi.org/10.1016/j.msec.2016.08.045
158. Jeong HJ, Gwak SJ, Seo KD, et al., 2020, Fabrication of three-dimensional composite scaffold for simultaneous alveolar bone regeneration in dental implant installation. Int J Mol Sci, 21(5):1863. https://doi.org/10.3390/ijms21051863
159. Zhao M, Yang D, Fan S, et al., 2022, 3D-printed strong dental crown with multi-scale ordered architecture, high-precision, and bioactivity. Adv Sci (Weinh) 9:e2104001. https://doi.org/10.1002/advs.202104001
160. Mai HN, Lee KB, Lee DH, 2017, Fit of interim crowns fabricated using photopolymer-jetting 3D printing. J Prosthet Dent, 118:208–215. https://doi.org/10.1016/j.prosdent.2016.10.030
161. Park CH, Rios HF, Jin Q, et al., 2010, Biomimetic hybrid scaffolds for engineering human tooth-ligament interfaces. Biomaterials, 31:5945–5952. https://doi.org/10.1016/j.biomaterials.2010.04.027
162. Khorsandi D, Fahimipour A, Abasian P, et al., 2021, 3D and 4D printing in dentistry and maxillofacial surgery: Printing techniques, materials, and applications. Acta Biomater, 122:26–49. https://doi.org/10.1016/j.actbio.2020.12.044
163. Dehurtevent M, Robberecht L, Hornez JC, et al., 2017, Stereolithography: A new method for processing dental ceramics by additive computer-aided manufacturing. Dent Mater, 33:477–485. https://doi.org/10.1016/j.dental.2017.01.018
164. Deng K, Chen H, Wei W, et al., 2023, Accuracy of tooth positioning in 3D-printing aided manufactured complete dentures: An in vitro study. J Dent, 104459. https://doi.org/https://doi.org/10.1016/j.jdent.2023.104459
165. Baijens LW, Clavé P, Cras P, et al., 2016, European Society for Swallowing Disorders European Union Geriatric Medicine Society white paper: Oropharyngeal dysphagia as a geriatric syndrome. Clin Interv Aging, 11:1403–1428. https://doi.org/10.2147/cia.S107750
166. Burgos R, Bretón I, Cereda E, et al., 2018, ESPEN guideline clinical nutrition in neurology. Clin Nutr, 37:354–396. https://doi.org/10.1016/j.clnu.2017.09.003
167. Chua CK, Yeong WY, Tan HW, et al., 2022, Pant A: Digital Gastronomy. World Scientific, 4.
168. Liu Z, Bhandari B, Guo C, et al., 2021, 3D printing of shiitake mushroom incorporated with gums as dysphagia diet. Foods, 10(9):2189. https://doi.org/10.3390/foods10092189
169. Lee JH, Won DJ, Kim HW, et al., 2019, Effect of particle size on 3D printing performance of the food-ink system with cellular food materials. J Food Eng, 256:1–8. https://doi.org/10.1016/j.jfoodeng.2019.03.014
170. Pant A, Lee AY, Karyappa R, et al., 2021, 3D food printing of fresh vegetables using food hydrocolloids for dysphagic patients. Food Hydrocolloids, 114:106546. https://doi.org/10.1016/j.foodhyd.2020.106546
171. Zhang Y, Lee AY, Pojchanun K, et al., 2022, Systematic engineering approach for optimization of multi-component alternative protein-fortified 3D printing food Ink. Food Hydrocolloids, 131:107803. https://doi.org/10.1016/j.foodhyd.2022.107803
172. Lee AY, Pant A, Pojchanun K, et al., 2021, Three-dimensional printing of food foams stabilized by hydrocolloids for hydration in dysphagia. Int J Bioprint, 7:156–168. https://doi.org/10.18063/ijb.v7i4.393
173. Dick A, Bhandari B, Dong XP, et al., 2020, Feasibility study of hydrocolloid incorporated 3D printed pork as dysphagia food. Food Hydrocolloids, 107:105940. https://doi.org/10.1016/j.foodhyd.2020.105940