Biomechanical evaluation of an osteoporotic anatomical 3D printed posterior lumbar interbody fusion cage with internal lattice design based on weighted topology optimization
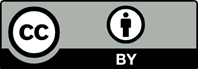
In this study, we designed and manufactured a posterior lumbar interbody fusion cage for osteoporosis patients using 3D-printing. The cage structure conforms to the anatomical endplate’s curved surface for stress transmission and internal lattice design for bone growth. Finite element (FE) analysis and weight topology optimization under different lumbar spine activity ratios were integrated to design the curved surface (CS-type) cage using the endplate surface morphology statistical results from the osteoporosis patients. The CS-type and plate (P-type) cage biomechanical behaviors under different daily activities were compared by performing non-linear FE analysis. A gyroid lattice with 0.25 spiral wall thickness was then designed in the internal cavity of the CS-type cage. The CS-cage was manufactured using metal 3D printing to conduct in vitro biomechanical tests. The FE analysis result showed that the maximum stress values at the inferior L3 and superior L4 endplates under all daily activities for the P-type cage implantation model were all higher than those for the CS-type cage. Fracture might occur in the P-type cage because the maximum stresses found in the endplates exceeded its ultimate strength (about 10 MPa) under flexion, torsion and bending loads. The yield load and stiffness of our designed CS-type cage fall into the optional acceptance criteria for the ISO 23089 standard under all load conditions. This study approved a posterior lumbar interbody fusion cage designed to have osteoporosis anatomical curved surface with internal lattice that can achieve appropriate structural strength, better stress transmission between the endplate and cage, and biomechanically tested strength that meets the standard requirements for marketed cages.
1. Zhenjun Z, Fogel GR, Liao Z, et al., 2018, Biomechanical analysis of lateral lumbar interbody fusion constructs with various fixation options: Based on a validated finite element model. World Neurosurg, 114: e1120–e1129. https://doi.org/10.1016/j.wneu.2018.03.158
2. Zhong ZC, Wei SH, Wang JP, et al., 2006, Finite element analysis of the lumbar spine with a new cage using a topology optimization method. Med Eng Phys, 28:90–98. https://doi.org/10.1016/j.medengphy.2005.03.007
3. Zhang F, Xu HC, Yin B, et al., 2016, Can an Endplate-conformed cervical cage provide a better biomechanical environment than a typical non-conformed cage? A finite element model and cadaver study. Orthop Surg, 8: 367–376. https://doi.org/10.1111/os.12261
4. Hakato J, Pezowicz C, Wronski J, et al., 2007, The process of subsidence after cervical stabilizations by cage alone, cage with plate and plate-cage. A biomechanical comparative study. Neurol Neurochir Pol, 41: 411–416.
5. Patel SP, Lee JJ, Hecht GG, et al., 2016, Normative Vertebral Hounsfield unit values and correlation with bone mineral density. J Clin Exp Orthop, 2: 14. https://doi.org/10.4172/2471-8416.100014
6. Griffith JF, Leung PC, Lee R, et al., 2009, Effect of osteoporosis on morphology and mobility of the lumbar spine. Spine (Phila Pa 1976), 34: E115–E121. https://doi.org/10.1097/brs.0b013e3181895aca
7. Tosun Ö, Fidan F, Erdil F, et al., 2012, Assessment of lumbar vertebrae morphology by magnetic resonance imaging in osteoporosis. Skeletal Radiol, 41: 1583–1590. https://doi.org/10.1007/s00256-012-1435-0
8. Le TV, Baaj AA, Dakwar E, et al., 2012, Subsidence of polyetheretherketone intervertebral cages in minimally invasive lateral retroperitoneal transpsoas lumbar interbody fusion. Spine (Phila Pa 1976), 37: 1268–1273. https://doi.org/10.1097/brs.0b013e3182458b2f
9. Taniguchi N, Fujibayashi S, Takemoto M, et al., 2016, Effect of pore size on bone ingrowth into porous titanium implants fabricated by additive manufacturing: An in vivo experiment. Mater Sci Eng C Mater Biol Appl, 59: 690–701. https://doi.org/10.1016/j.msec.2015.10.069
10. Yang J, Cai H, Lv J, et al., 2014, In vivo study of a self-stabilizing artificial vertebral body fabricated by electron beam melting. Spine (Phila Pa 1976), 39: E486–E492. https://doi.org/10.1097/BRS.0000000000000211
11. Wu SH, Li Y, Zhang YQ, et al., 2013, Porous titanium-6 aluminum-4 vanadium cage has better osseointegration and less micromotion than a poly-ether-ether-ketone cage in sheep vertebral fusion. Artif Organs, 37: E191–E201. https://doi.org/10.1111/aor.12153
12. Li F, Li J, Xu G, et al., 2015, Fabrication, pore structure and compressive behavior of anisotropic porous titanium for human trabecular bone implant applications. J Mech Behav Biomed Mater,46: 104–114. https://doi.org/10.1016/j.jmbbm.2015.02.023
13. Chang B, Song W, Han T, et al., 2016, Influence of pore size of porous titanium fabricated by vacuum diffusion bonding of titanium meshes on cell penetration and bone ingrowth. Acta Biomater, 33: 311–321. https://doi.org/10.1016/j.actbio.2016.01.022
14. Liao CY, Chien CL, Pu TW, et al., 2022, Assessment of lumbar vertebrae morphology by computed tomography in older adults with osteoporosis. Current Medical Imaging, 18: 1195–1203. https://doi.org/10.2174/1573405618666220404160213
15. Chazal J, Tanguy A, Bourges M, et al., 1985, Biomechanical properties of spinal ligaments and a histological study of the supraspinal ligament in traction. J Biomech, 18: 167–176. https://doi.org/10.1016/0021-9290(85)90202-7
16. Xiao Z, Wang L, Gong H, et al., 2011, A non-linear finite element model of human L4-L5 lumbar spinal segment with three dimensional solid element ligaments. Theoretical and Applied Mechanics Letters, 1: 064001. https://doi.org/10.1063/2.1106401
17. Yamamoto I, Panjabi MM, Crisco T, et al., 1989, Three-dimensional movements of the whole lumbar spine and lumbosacral joint. Spine (Phila Pa 1976), 14.11:1256–1260. https://doi.org/10.1097/00007632-198911000-00020
18. Schmoelz W, Huber JF, Nydegger T, et al., 2003, Dynamic stabilization of the lumbar spine and its effects on adjacent segments: An in vitro experiment. J Spinal Disord Tech, 16: 418–423. https://doi.org/10.1097/00024720-200308000-00015
19. Chen SH, Tai CL, Lin CY, et al., 2008, Biomechanical comparison of a new stand-alone anterior lumbar interbody fusion cage with established fixation techniques-a three-dimensional finite element analysis. BMC Musculoskelet Disord, 9: 88. https://doi.org/10.1186/1471-2474-9-88
20. Li CH, Wu CH, Lin CL, 2020, Design of a patient-specific mandible reconstruction implant with dental prosthesis for metal 3D printing using integrated weighted topology optimization and finite element analysis. J Mech Behav Biomed Mater, 105: 103700. https://doi.org/10.1016/j.jmbbm.2020.103700
21. Cobian D, Heiderscheit B, Daehn N, et al., 2011, Comparison of daily motion of the cervical and lumbar spine to ASTM F2423-11 and ISO 18192-1.2011 standard testing. J ASTM Int, 9: 1–10. https://doi.org/10.1520/JAI103522
22. Pflugmacher R, Schleicher P, Gumnior S, et al., 2004, Biomechanical comparison of bioabsorbable cervical spine interbody fusion cages. Spine (Phila Pa 1976), 29: 1717–1722. https://doi.org/10.1097/01.brs.0000134565.17078.4C
23. Tan JS, Bailey CS, Dvorak MF, et al., 2005, Interbody device shape and size are important to strengthen the vertebra-implant interface. Spine (Phila Pa 1976), 30:638–644. https://doi.org/10.1097/01.brs.0000155419.24198.35
24. Machinery for Forestry-forwarders-terms, Definitions and Commercial Specifications. ISO 13860.