The biological properties of 3D-printed degradable magnesium alloy WE43 porous scaffolds via the oxidative heat strategy
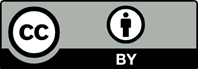
As a biodegradable material, magnesium alloy has a modulus similar to that of bone, and given the biological activity of its degradation products, it has the potential to be a bone grafting material. Oxidation heat treatment is a very effective passivation method that may reduce the rate of magnesium alloy degradation. Oxidation heat treatment increases the rare earth oxide content of the scaffold as well as the corrosion resistance of the scaffold. The overall cytotoxicity of the as-printed scaffolds (APSs) and oxidation heat-treated scaffolds (OHSs) showed that OHSs accelerated cell proliferation. In the apoptosis experiment, the OHS group had a cell survival rate between that of the control group and of the as-printed group. In the osteogenic induction experiment, the alkaline phosphatase activity and the quantity of mineralized nodules were greater in the APS and OHS groups than in the control group. Marker proteins for bone growth were expressed at higher levels in the APS and OHS groups than in the control group. Therefore, oxidation heat-treated 3D printing scaffolds with good biocompatibility and osteogenic properties have great potential to be made into advanced biomaterials that can be used to fix bone defects.
1. Archunan MW, Petronis S, 2021, Bone grafts in trauma and orthopaedics. Cureus, 13(9):e17705. https://doi.org/10.7759/cureus.17705
2. Grambart ST, Anderson DS, Anderson TD, 2020, Bone grafting options. Clin Podiatr Med Surg, 37(3):593–600. https://doi.org/10.1016/j.cpm.2020.03.012
3. Šupová M, 2015, Substituted hydroxyapatites for biomedical applications: A review. Ceram Int, 41(8):9203–9231. https://doi.org/10.1016/j.ceramint.2015.03.316
4. Kani KK, Porrino JA, Chew FS, 2020, External fixators: Looking beyond the hardware maze. Skelet Radiol, 49(3):359–374. https://doi.org/10.1007/s00256-019-03306-w
5. Yi-Wu J, Hsieh CH, Lin ZY, 2022, Novel high-speed 3D printing method using selective oil sintering with thermoplastic polyurethane powder printing. Int J Bioprint, 8(2):521. https://doi.org/10.18063/ijb.v8i2.521
6. Yin C, Zhang T, Wei Q, et al., 2022, Surface treatment of 3D printed porous Ti6Al4V implants by ultraviolet photofunctionalization for improved osseointegration. Bioactive Mater, 7:26–38. https://doi.org/10.1016/j.bioactmat.2021.05.043
7. Kadakia RJ, Wixted CM, Allen NB, et al., 2020, Clinical applications of custom 3D printed implants in complex lower extremity reconstruction. 3D Print Med, 6(1):29. https://doi.org/10.1186/s41205-020-00083-4
8. Deng F, Liu L, Li Z, et al., 2021, 3D printed Ti6Al4V bone scaffolds with different pore structure effects on bone ingrowth. J Biol Eng, 15(1):4. https://doi.org/10.1186/s13036-021-00255-8
9. Sumner DR, 2015, Long-term implant fixation and stress-shielding in total hip replacement. J Biomech, 48(5):797-800. https://doi.org/10.1016/j.jbiomech.2014.12.021
10. Biber R, Pauser J, Gesslein M, et al., 2016, Magnesium-based absorbable metal screws for intra-articular fracture fixation. Case Rep Orthop, 2016:9673174. https://doi.org/10.1155/2016/9673174
11. Leem YH, Lee KS, Kim JH, et al., 2016, Magnesium ions facilitate integrin alpha 2- and alpha 3-mediated proliferation and enhance alkaline phosphatase expression and activity in hBMSCs. J Tissue Eng Regen Med, 10(10):E527–E536. https://doi.org/10.1002/term.1861
12. Chen K, Xie X, Tang H, et al., 2020, In vitro and in vivo degradation behavior of Mg-2Sr-Ca and Mg-2Sr-Zn alloys. Bioact Mater, 5(2):275–285. https://doi.org/10.1016/j.bioactmat.2020.02.014
13. Xia D, Liu Y, Wang S, et al., 2018, In vitro and in vivo investigation on biodegradable Mg-Li-Ca alloys for bone implant application. Sci China Mater, 62(2):256–272. https://doi.org/10.1007/s40843-018-9293-8
14. Li Z, Gu X, Lou S, et al., 2008, The development of binary Mg–Ca alloys for use as biodegradable materials within bone. Biomaterials, 29(10):1329–1344. https://doi.org/10.1016/j.biomaterials.2007.12.021
15. He LY, Zhang XM, Liu B, et al., 2016, Effect of magnesium ion on human osteoblast activity. Braz J Med Biol Res, 49(7): e5257. https://doi.org/10.1590/1414-431X20165257
16. Yang Y, He C, Dianyu E, et al., 2020, Mg bone implant: Features, developments and perspectives. Mater Design, 185:108259. https://doi.org/10.1016/j.matdes.2019.108259
17. Jiang Q, Lu D, Liu C, et al., 2021, The Pilling-Bedworth ratio of oxides formed from the precipitated phases in magnesium alloys. Front Mater, 8:761052. https://doi.org/10.3389/fmats.2021.761052
18. Kim YM, Yim CD, Kim HS, et al., 2011, Key factor influencing the ignition resistance of magnesium alloys at elevated temperatures. Script Mater, 65(11):958–961. https://doi.org/10.1016/j.scriptamat.2011.08.019
19. Wu Y, Wang YM, Zhao DW, et al., 2019, In vivo study of microarc oxidation coated Mg alloy as a substitute for bone defect repairing: Degradation behavior, mechanical properties, and bone response. Colloids Surf B Biointerfaces, 181:349–359. https://doi.org/10.1016/j.colsurfb.2019.05.052
20. Lalk M, Reifenrath J, Angrisani N, et al., 2013, Fluoride and calcium-phosphate coated sponges of the magnesium alloy AX30 as bone grafts: A comparative study in rabbits. J Mater Sci Mater Med, 24(2):417–436. https://doi.org/10.1007/s10856-012-4812-2
21. Liu J, Yin B, Song F, et al., 2022, Improving corrosion resistance of additively manufactured WE43 magnesium alloy by high temperature oxidation for biodegradable applications. J Magnes Alloys. https://doi.org/10.1016/j.jma.2022.08.009
22. Bar F, Berger L, Jauer L, et al., 2019, Laser additive manufacturing of biodegradable magnesium alloy WE43: A detailed microstructure analysis. Acta Biomater, 98:36–49. https://doi.org/10.1016/j.actbio.2019.05.056
23. Liu J, Liu B, Min S, et al., 2022, Biodegradable magnesium alloy WE43 porous scaffolds fabricated by laser powder bed fusion for orthopedic applications: Process optimization, in vitro and in vivo investigation. Bioact Mater, 16:301–319. https://doi.org/10.1016/j.bioactmat.2022.02.020
24. Zhang Q, Li Q, Chen X, 2020, Effect of heat treatment on corrosion behavior of Mg–5Gd–3Y–0.5Zr alloy. RSC Adv, 10(71):43371–43382. https://doi.org/10.1039/d0ra08933h
25. Lu WC, Pringa E, Chou L, 2017, Effect of magnesium on the osteogenesis of normal human osteoblasts. Magnes Res, 30(2):42–52. https://doi.org/10.1684/mrh.2017.0422
26. Feyerabend F, Fischer J, Holtz J, et al., 2010, Evaluation of short-term effects of rare earth and other elements used in magnesium alloys on primary cells and cell lines. Acta Biomater, 6(5):1834–1842. https://doi.org/10.1016/j.actbio.2009.09.024
27. Li F, Gong A, Qiu L, et al., 2017, Simultaneous determination of trace rare-earth elements in simulated water samples using ICP-OES with TODGA extraction/back-extraction. PLoS One, 12(9):e0185302. https://doi.org/10.1371/journal.pone.0185302
28. Vimalraj S, 2020, Alkaline phosphatase: Structure, expression and its function in bone mineralization. Gene, 754:144855. https://doi.org/10.1016/j.gene.2020.144855
29. Prins HJ, Braat AK, Gawlitta D, et al., 2014, In vitro induction of alkaline phosphatase levels predicts in vivo bone forming capacity of human bone marrow stromal cells. Stem Cell Res, 12(2):428–440. https://doi.org/10.1016/j.scr.2013.12.001
30. Dubey N, Bentini R, Islam I, et al., 2015, Graphene: A versatile carbon-based material for bone tissue engineering. Stem Cells Int, 2015:804213. https://doi.org/10.1155/2015/804213
31. Komori T, 2009, Regulation of osteoblast differentiation by Runx2. Osteoimmunology. Adv Exp Med Biol, 658:43–49.
32. Arumugam B, Vishal M, Shreya S, et al., 2019, Parathyroid hormone-stimulation of Runx2 during osteoblast differentiation via the regulation of lnc-SUPT3H-1:16 (RUNX2-AS1:32) and miR-6797-5p. Biochimie, 158:43-52. https://doi.org/10.1016/j.biochi.2018.12.006
33. Qiao M, Shapiro P, Fosbrink M, et al., 2006, Cell cycle-dependent phosphorylation of the RUNX2 transcription factor by cdc2 regulates endothelial cell proliferation. J Biol Chem, 281(11):7118–7128. https://doi.org/10.1074/jbc.M508162200
34. San Martin IA, Varela N, Gaete M, et al., 2009, Impaired cell cycle regulation of the osteoblast-related heterodimeric transcription factor Runx2-Cbfbeta in osteosarcoma cells. J Cell Physiol, 221(3):560–571. https://doi.org/10.1002/jcp.21894
35. Galindo M, Pratap J, Young DW, et al., 2005, The bone-specific expression of Runx2 oscillates during the cell cycle to support a G1-related antiproliferative function in osteoblasts. J Biol Chem, 280(21):20274–20285. https://doi.org/10.1074/jbc.M413665200
36. Sinha KM, Zhou X, 2013, Genetic and molecular control of osterix in skeletal formation. J Cell Biochem, 114(5):975–984. https://doi.org/10.1002/jcb.24439
37. Nakashima K, Zhou X, Kunkel G, et al., 2002, The novel zinc finger-containing transcription factor osterix is required for osteoblast differentiation and bone formation. Cell, 108(1):17–29. https://doi.org/10.1016/s0092-8674(01)00622-5
38. Jun JH, Yoon WJ, Seo SB, et al., 2010, BMP2-activated Erk/MAP kinase stabilizes Runx2 by increasing p300 levels and histone acetyltransferase activity. J Biol Chem, 285(47):36410–36419. https://doi.org/10.1074/jbc.M110.142307
39. Yang Y, Rao J, Liu H, et al., 2022, Biomimicking design of artificial periosteum for promoting bone healing. J Orthop Transl, 36:18–32. https://doi.org/10.1016/j.jot.2022.05.013