Engineered extracellular vesicle-mediated delivery of miR-199a-3p increases the viability of 3D-printed cardiac patches
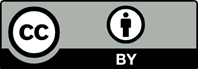
In recent years, extrusion-based three-dimensional (3D) bioprinting is employed for engineering cardiac patches (CP) due to its ability to assemble complex structures from hydrogel-based bioinks. However, the cell viability in such CPs is low due to shear forces applied on the cells in the bioink, inducing cellular apoptosis. Herein, we investigated whether the incorporation of extracellular vesicles (EVs) in the bioink, engineered to continually deliver the cell survival factor miR-199a-3p would increase the viability within the CP. EVs from THP-1-derived activated macrophages (MΦ) were isolated and characterized by nanoparticle tracking analysis (NTA), cryogenic electron microscopy (cryo-TEM), and Western blot analysis. MiR-199a-3p mimic was loaded into EVs by electroporation after optimization of applied voltage and pulses. Functionality of the engineered EVs was assessed in neonatal rat cardiomyocyte (NRCM) monolayers using immunostaining for the proliferation markers ki67 and Aurora B kinase. To examine the effect of engineered EVs on 3D-bioprinted CP viability, the EVs were added to the bioink, consisting of alginate-RGD, gelatin, and NRCM. Metabolic activity and expression levels of activated-caspase 3 for apoptosis of the 3D-bioprinted CP were evaluated after 5 days. Electroporation (850 V with 5 pulses) was found to be optimal for miR loading; miR-199a-3p levels in EVs increased fivefold compared to simple incubation, with a loading efficiency of 21.0%. EV size and integrity were maintained under these conditions. Cellular uptake of engineered EVs by NRCM was validated, as 58% of cTnT+ cells internalized EVs after 24 h. The engineered EVs induced CM proliferation, increasing the ratio of cell-cycle re-entry of cTnT+ cells by 30% (Ki67) and midbodies+ cell ratio by twofold (Aurora B) compared with the controls. The inclusion of engineered EVs in bioink yielded CP with threefold greater cell viability compared to bioink with no EVs. The prolonged effect of EVs was evident as the CP exhibited elevated metabolic activities after 5 days, with less apoptotic cells compared to CP with no EVs. The addition of miR-199a-3p–loaded EVs to the bioink improved the viability of 3D-printed CP and is expected to contribute to their integration in vivo.
1. Laflamme MA, Murry CE, 2011, Heart regeneration. Nature, 473(7347):326.
2. Laflamme MA, Murry CE, 2005, Regenerating the heart. Nat Biotechnol, 23:845.
3. Liau B, Christoforou N, Leong KW, et al., 2011, Pluripotent stem cell-derived cardiac tissue patch with advanced structure and function. Biomaterials, 32(35):9180–9187.
4. Bar A, Cohen S, 2020, Inducing endogenous cardiac regeneration: Can biomaterials connect the dots? Front Bioeng Biotechnol, 8:126.
5. Tiburcy M, Hudson JE, Balfanz P, et al.2017, Defined engineered human myocardium with advanced maturation for applications in heart failure modeling and repair. Circulation, 135(19):1832–1847.
6. Jabbour RJ, Owen TJ, Pandey P, et al., 2021, In vivo grafting of large engineered heart tissue patches for cardiac repair. JCI Insight, 6(15):e144068.
7. Gao L, Gregorich ZR, Wuqiang Z, et al., 2018, Large cardiac muscle patches engineered from human induced-pluripotent stem cell–derived cardiac cells improve recovery from myocardial infarction in swine. Circulation, 137(16):1712–1730.
8. Riegler J, Tiburcy M, Ebert A, et al.2015, Human engineered heart muscles engraft and survive long term in a rodent myocardial infarction model. Circ Res, 117(8):720–730.
9. Shadrin IY, Allen BW, Qian Y, et al., 2017, Cardiopatch platform enables maturation and scale-up of human pluripotent stem cell-derived engineered heart tissues. Nat Commun, 8(1):1825.
10. Fleischer S, Feiner R, Dvir T, 2017, Cutting-edge platforms in cardiac tissue engineering. Curr Opin Biotechnol, 47:23–29.
11. Lee A, Hudson AR, Shiwarski DJ, et al., 2019, 3D bioprinting of collagen to rebuild components of the human heart. Science (80- ), 365(6452):482–487.
12. Bejleri D, Streeter BW, Nachlas ALY, et al., 2018, A bioprinted cardiac patch composed of cardiac-specific extracellular matrix and progenitor cells for heart repair. Adv Healthc Mater, 7(23):1800672.
13. Skardal A, Devarasetty M, Kang HW, et al., 2015, A hydrogel bioink toolkit for mimicking native tissue biochemical and mechanical properties in bioprinted tissue constructs. Acta Biomater, 25:24–34.
14. Placone JK, Engler AJ, 2018, Recent advances in extrusion‐based 3D printing for biomedical applications. Adv Healthc Mater, 7(8):1701161.
15. Koti P, Muselimyan N, Mirdamadi E, et al., 2019. Use of GelMA for 3D printing of cardiac myocytes and fibroblasts. J 3D Print Med, 3(1):11–22.
16. Das S, Kim SW, Choi YJ, et al., 2019, Decellularized extracellular matrix bioinks and the external stimuli to enhance cardiac tissue development in vitro. Acta Biomater, 95:188–200.
17. Malekpour A, Chen X, 2022, Printability and cell viability in extrusion-based bioprinting from experimental, computational, and machine learning views. J Funct Biomater, 13(2):40.
18. Ruvinov E, Leor J, Cohen S, 2011, The promotion of myocardial repair by the sequential delivery of IGF-1 and HGF from an injectable alginate biomaterial in a model of acute myocardial infarction. Biomaterials, 32(2):565–578.
19. Bejerano T, Etzion S, Elyagon S, et al., 2018, Nanoparticle delivery of miRNA-21 mimic to cardiac macrophages improves myocardial remodeling after myocardial infarction. Nano Lett, 18(9):5885–5891.
20. Goldshtein M, Shamir S, Vinogradov E, et al., 2019, Co-assembled Ca2+ alginate-sulfate nanoparticles for intracellular plasmid DNA delivery. Mol Ther - Nucleic Acids, 16:378–390.
21. Oduk Y, Zhu W, Kannappan R, et al., 2018, VEGF nanoparticles repair the heart after myocardial infarction. Am J Physiol Heart Circ Physiol, 314(2):H278–H284.
22. Liu B, Lee BW, Nakanishi K, et al., 2018, Cardiac recovery via extended cell-free delivery of extracellular vesicles secreted by cardiomyocytes derived from induced pluripotent stem cells. Nat Biomed Eng, 2(5):293.
23. Wang LL, Liu Y, Chung JJ, et al., 2017, Sustained miRNA delivery from an injectable hydrogel promotes cardiomyocyte proliferation and functional regeneration after ischaemic injury. Nat Biomed Eng, 1(12):983.
24. Montgomery RL, van Rooij E, 2011, Therapeutic advances in microRNA targeting. J Cardiovasc Pharmacol, 57(1):1–7.
25. Muthiah M, Park IK, Cho CS, 2013, Nanoparticle-mediated delivery of therapeutic genes: Focus on miRNA therapeutics. Expert Opin Drug Deliv [Internet], 10(9):1259–1273. https://doi.org/10.1517/17425247.2013.798640
26. Barwari T, Joshi A, Mayr M, 2016, MicroRNAs in cardiovascular disease. J Am Coll Cardiol, 68(23):2577–2584.
27. Eulalio A, Mano M, Dal Ferro M, et al., 2012, Functional screening identifies miRNAs inducing cardiac regeneration. Nature, 492(7429):376.
28. Torrini C, Cubero RJ, Dirkx E, et al., 2019, Common regulatory pathways mediate activity of microRNAs inducing cardiomyocyte proliferation. Cell Rep, 27(9):2759– 2771.
29. Shatseva T, Lee DY, Deng Z, et al., 2011, MicroRNA miR- 199a-3p regulates cell proliferation and survival by targeting caveolin-2. J Cell Sci, 124(16):2826 LP – 2836.
30. Balaj L, Atai NA, Chen W, et al., 2015, Heparin affinity purification of extracellular vesicles. Sci Rep [Internet], 5:10266. Available from: https://www.ncbi.nlm.nih.gov/ pubmed/25988257
31. Simons M, Raposo G, 2009, Exosomes–vesicular carriers for intercellular communication. Curr Opin Cell Biol, 21(4):575–581.
32. Webber J, Steadman R, Mason MD, et al., 2010, Cancer exosomes trigger fibroblast to myofibroblast differentiation. Cancer Res, 70(23):9621–9630.
33. Costa-Silva B, Aiello NM, Ocean AJ, et al., 2015, Pancreatic cancer exosomes initiate pre-metastatic niche formation in the liver. Nat Cell Biol, 17(6):816.
34. Emanueli C, Shearn AIU, Angelini GD, et al., 2015, Exosomes and exosomal miRNAs in cardiovascular protection and repair. Vasc Pharmacol, 71:24–30.
35. Zhang Y, Liu D, Chen X, et al., 2010, Secreted monocytic miR-150 enhances targeted endothelial cell migration. Mol Cell, 39(1):133–144.
36. Arslan F, Lai RC, Smeets MB, et al., 2013, Mesenchymal stem cell-derived exosomes increase ATP levels, decrease oxidative stress and activate PI3K/Akt pathway to enhance myocardial viability and prevent adverse remodeling after myocardial ischemia/reperfusion injury. Stem Cell Res, 10(3):301–312.
37. Ferguson SW, Wang J, Lee CJ, et al., 2018, The microRNA regulatory landscape of MSC-derived exosomes: A systems view. Sci Rep, 8(1):1419.
38. Wu R, Gao W, Yao K, et al., 2019, Roles of exosomes derived from immune cells in cardiovascular diseases. Front Immunol, 10:648.
39. Zudaire E, Gambardella L, Kurcz C, et al., 2011, A computational tool for quantitative analysis of vascular networks. PLoS One, 6(11):e27385.
40. Bondalapati S, Ruvinov E, Kryukov O, et al., 2014, Rapid end‐group modification of polysaccharides for biomaterial applications in regenerative medicine. Macromol Rapid Commun, 35(20):1754–1762.
41. Hinton TJ, Jallerat Q, Palchesko RN, et al., 2015, Three-dimensional printing of complex biological structures by freeform reversible embedding of suspended hydrogels. Sci Adv, 1(9):e1500758.
42. Ouyang L, Yao R, Zhao Y, et al., 2016, Effect of bioink properties on printability and cell viability for 3D bioplotting of embryonic stem cells. Biofabrication [Internet], 8(3):35020. https://doi.org/10.1088/1758-5090/8/3/035020
43. Kowal J, Tkach M, Théry C, 2014, Biogenesis and secretion of exosomes. Curr Opin Cell Biol, 29:116–125.
44. Xi XM, Xia SJ, Lu R, 2021, Drug loading techniques for exosome-based drug delivery systems. Die Pharm Int J Pharm Sci, 76(2–3):61–67.
45. Fu S, Wang Y, Xia X, et al., 2020, Exosome engineering: Current progress in cargo loading and targeted delivery. NanoImpact, 20:100261.
46. Pomatto MAC, Bussolati B, D’Antico S, et al., 2019, Improved loading of plasma-derived extracellular vesicles to encapsulate antitumor miRNAs. Mol Ther Clin Dev, 13:133–144.
47. Perbellini F, Watson SA, Bardi I, et al., 2018, Heterocellularity and cellular cross-talk in the cardiovascular system. Front Cardiovasc Med, 5:143.
48. Whitehead AJ, Engler AJ, 2021, Regenerative cross talk between cardiac cells and macrophages. Am J Physiol Circ Physiol, 320(6):H2211–H2221.
49. Honold L, Nahrendorf M, 2018, Resident and monocyte-derived macrophages in cardiovascular disease. Circ Res, 122(1):113–127.
50. Wang Y, Zhao M, Liu S, et al., 2020, Macrophage-derived extracellular vesicles: Diverse mediators of pathology and therapeutics in multiple diseases. Cell Death Dis, 11(10):924.
51. Hausser J, Syed AP, Selevsek N, et al., 2013, Timescales and bottlenecks in miRNA‐dependent gene regulation. Mol Syst Biol, 9(1):711.
52. Zhang Z, Qin YW, Brewer G, et al., 2012, MicroRNA degradation and turnover: Regulating the regulators. Wiley Interdiscip Rev RNA, 3(4):593–600.
53. Uygur A, Lee RT, 2016, Mechanisms of cardiac regeneration. Dev Cell, 36(4):362–374.
54. Gangadaran P, Rajendran RL, Oh JM, et al., 2020, Extracellular vesicles derived from macrophage promote angiogenesis in vitro and accelerate new vasculature formation in vivo. Exp Cell Res, 394(2):112146.
55. Hosseinkhani B, Kuypers S, van den Akker NMS, et al., 2018, Extracellular vesicles work as a functional inflammatory mediator between vascular endothelial cells and immune cells. Front Immunol, 9:1789.
56. Sapir Y, Kryukov O, Cohen S, 2011, Integration of multiple cell-matrix interactions into alginate scaffolds for promoting cardiac tissue regeneration. Biomaterials, 32(7):1838–1847.
57. Wang C, Zhang C, Liu L, et al., 2017, Macrophage-derived mir-155-containing exosomes suppress fibroblast proliferation and promote fibroblast inflammation during cardiac injury. Mol Ther, 25(1):192–204.
58. Kishore R, Khan M, 2016, More than tiny sacks: Stem cell exosomes as cell-free modality for cardiac repair. Circ Res, 118(2):330–343.
59. Lennaárd AJ, Mamand DR, Wiklander RJ, et al., 2022, Optimised electroporation for loading of extracellular vesicles with doxorubicin. Pharmaceutics, 14(1):38.
60. Roche CD, Sharma P, Ashton AW, et al., 2021, Printability, durability, contractility and vascular network formation in 3D bioprinted cardiac endothelial cells using alginate– gelatin hydrogels. Front Bioeng Biotechnol, 9:636257.
61. Ouyang L, Yao R, Mao S, et al., 2015, Three-dimensional bioprinting of embryonic stem cells directs highly uniform embryoid body formation. Biofabrication, 7(4):44101.
62. Shapira A, Noor N, Asulin M, et al., 2018, Stabilization strategies in extrusion-based 3D bioprinting for tissue engineering. Appl Phys Rev, 5(4):41112.
63. Querdel E, Reinsch M, Castro L, et al., 2021, Human engineered heart tissue patches remuscularize the injured heart in a dose-dependent manner. Circulation, 143(20):1991–2006.