A holistic model for melt electrowritten threedimensional structured materials based on residual charge
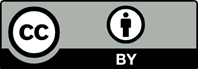
The printing accuracy of polymer melt electrowriting is adversely affected by the residual charge entrapped within the fibers, especially for three-dimensional (3D) structured materials or multilayered scaffolds with small interfiber distances. To clarify this effect, an analytical charge-based model is proposed herein. The electric potential energy of the jet segment is calculated considering the amount and distribution of the residual charge in the jet segment and the deposited fibers. As the jet deposition proceeds, the energy surface assumes different patterns, which constitute different modes of evolution. The manner in which the various identified parameters affect the mode of evolution are represented by three charge effects, including the global, local, and polarization effect. Based on these representations, typical modes of energy surface evolution are identified. Moreover, the lateral characteristic curve and characteristic surface are advanced to analyze the complex interplay between fiber morphologies and residual charge. Different parameters contribute to this interplay either by affecting residual charge, fiber morphologies, or the three charge effects. To validate this model, the effects of lateral location and grid number (i.e., number of fibers printed in each direction) on the fiber morphologies are investigated. Moreover, the “fiber bridging” phenomenon in parallel fiber printing is successfully explained. These results help to comprehensively understand the complex interplay between the fiber morphologies and the residual charge, thus furnishing a systematic workflow to improve printing accuracy.
1. Daghrery A, de Souza Araújo IJ, Castilho M, et al., 2022, Unveiling the potential of melt electrowriting in regenerative dental medicine. Acta Biomater, 20. https://doi.org/10.1016/j.actbio.2022.01.01
2. Li X, Liu B, Pei B, et al., 2020, Inkjet bioprinting of biomaterials. Chem Rev, 120: 10793–10833. https://doi.org/10.1021/acs.chemrev.0c00008
3. Jiang T, Munguia-Lopez JG, Flores-Torres S, et al., 2019, Extrusion bioprinting of soft materials: An emerging technique for biological model fabrication. Appl Phys Rev, 6: 011310. https://doi.org/10.1063/1.5059393
4. Ng WL, Lee JM, Zhou M, et al., 2019, Vat polymerization based bioprinting-process, materials, applications and regulatory challenges. Biofabrication, 12: 022011.
5. Saidy NT, Wolf F, Bas O, et al., 2019, biologically inspired scaffolds for heart valve tissue engineering via melt electrowriting. Small, 15: 1–15. https://doi.org/10.1002/smll.201900873
6. Boularaoui S, Al Hussein G, Khan KA, et al., 2020, An overview of extrusion-based bioprinting with a focus on induced shear stress and its effect on cell viability. Bioprinting, 20: e00093. https://doi.org/10.1016/j.bprint.2020.e00093
7. Mobaraki M, Ghaffari M, Yazdanpanah A, et al., 2020, Bioinks and bioprinting: A focused review. Bioprinting, 18: e00080. https://doi.org/10.1016/j.bprint.2020.e00080
8. Roseti L, Parisi V, Petretta M, et al., 2017, Scaffolds for bone tissue engineering: State of the art and new perspectives. Mater Sci Eng C, 78: 1246–1262. https://doi.org/10.1016/j.msec.2017.05.017
9. Kade JC, Dalton PD, 2021, Polymers for melt electrowriting. Adv Healthc Mater, 10: 202001232. https://doi.org/10.1002/adhm.202001232
10. Ko J, Mohtaram NK, Ahmed F, et al., 2014, Fabrication of poly (ε-caprolactone) microfiber scaffolds with varying topography and mechanical properties for stem cell-based tissue engineering applications. J Biomater Sci Polym Ed, 25: 1–17. https://doi.org/10.1080/09205063.2013.830913
11. Tourlomousis F, Ding H, Kalyon DM, et al., 2017, Melt electrospinning writing process guided by a “Printability Number.” J Manuf Sci Eng Trans ASME, 139: 081004. https://doi.org/10.1115/1.4036348
12. Dayan CB, Afghah F, Okan BS, et al., 2018, Modeling 3D melt electrospinning writing by response surface methodology. Mater Des, 148: 87–95. https://doi.org/10.1016/j.matdes.2018.03.053
13. Tourlomousis F, Jia C, Karydis T, et al., 2019, Machine learning metrology of cell confinement in melt electrowritten three-dimensional biomaterial substrates. Microsystems Nanoeng, 5: 15. https://doi.org/10.1038/s41378-019-0055-4
14. Peiffer QC, de Ruijter M, van Duijn J, et al., 2020, Melt electrowriting onto anatomically relevant biodegradable substrates: Resurfacing a diarthrodial joint. Mater Des, 195: 109025. https://doi.org/10.1016/j.matdes.2020.109025
15. Su Y, Zhang Z, Wan Y, et al., 2020, A hierarchically ordered compacted coil scaffold for tissue regeneration. NPG Asia Mater, 12: 4–13. https://doi.org/10.1038/s41427-020-0234-7
16. Jin Y, Gao Q, Xie C, et al., 2020, Fabrication of heterogeneous scaffolds using melt electrospinning writing: Design and optimization. Mater Des, 185: 108274. https://doi.org/10.1016/j.matdes.2019.108274
17. Hochleitner G, Chen F, Blum C, et al., 2018, Melt electrowriting below the critical translation speed to fabricate crimped elastomer scaffolds with non-linear extension behaviour mimicking that of ligaments and tendons. Acta Biomater, 72: 110–120. https://doi.org/10.1016/j.actbio.2018.03.023
18. Bas O, Angella DD, Baldwin JG, et al., 2017, An integrated design, material, and fabrication platform for engineering biomechanically and biologically functional soft tissues. ACS Appl Mater Interfaces, 9: 29430–29437. https://doi.org/10.1021/acsami.7b08617
19. Meng J, Boschetto F, Yagi S, et al., 2021, Design and manufacturing of 3D high-precision micro-fibrous poly (L-lactic acid) scaffold using melt electrowriting technique for bone tissue engineering. Mater Des, 210: 110063. https://doi.org/10.1016/j.matdes.2021.110063
20. Castilho M, Feyen D, Flandes-Iparraguirre M, et al., 2017, Melt electrospinning writing of poly-hydroxymethylglycolide-co-ε-caprolactone-based scaffolds for cardiac tissue engineering. Adv Healthc Mater, 6: 1700311. https://doi.org/10.1002/adhm.201700311
21. Ko J, Mohtaram NK, Lee PC, et al., 2015, Mathematical model for predicting topographical properties of poly (ε-caprolactone) melt electrospun scaffolds including the effects of temperature and linear transitional speed. J Micromechanics Microeng, 25: 045018. https://doi.org/10.1088/0960-1317/25/4/045018
22. Brooks-Richards TL, Paxton NC, Allenby MC, et al., 2022, Dissolvable 3D printed PVA moulds for melt electrowriting tubular scaffolds with patient-specific geometry. Mater Des, 215: 110466. https://doi.org/10.1016/j.matdes.2022.110466
23. Hochleitner G, Jüngst T, Brown TD, et al., 2015, Additive manufacturing of scaffolds with sub-micron filaments via melt electrospinning writing. Biofabrication, 7: 035002. https://doi.org/10.1088/1758-5090/7/3/035002
24. Hochleitner G, Hümmer JF, Luxenhofer R, et al., 2014, High definition fibrous poly(2-ethyl-2-oxazoline) scaffolds through melt electrospinning writing. Polymer (Guildf), 55: 5017–5023. https://doi.org/10.1016/j.polymer.2014.08.024
25. Collins G, Federici J, Imura Y, et al., 2012, Charge generation, charge transport, and residual charge in the electrospinning of polymers: A review of issues and complications, J Appl Phys, 111: 044701. https://doi.org/10.1063/1.3682464
26. Kim J, Bakirci E, Neill KLO, et al., 2021, Fiber bridging during melt electrowriting of poly (ε-Caprolactone) and the influence of fiber diameter and wall height. Macromol Mater Eng, 306: 2000685. https://doi.org/10.1002/mame.202000685
27. Ding H, Cao K, Zhang F, et al., 2019, A fundamental study of charge effects on melt electrowritten polymer fibers. Mater Des, 178: 107857. https://doi.org/10.1016/j.matdes.2019.107857
28. Wunner FM, Wille M, Noonan TG, et al., 2018, Melt electrospinning writing of highly ordered large volume scaffold architectures. Adv Mater, 30: 1706570. https://doi.org/10.1002/adma.201706570
29. He J, Hao G, Meng Z, et al., 2021, Expanding melt-based electrohydrodynamic printing of highly-ordered microfibrous architectures to cm-height via in situ charge neutralization. Adv Mater Technol, 7: 2101197. https://doi.org/10.1002/admt.202101197
30. Cao K, Zhang F, Zaeri A, et al., 2022, Quantitative investigation into the design and process parametric effects on the fiber‐entrapped residual charge for a polymer melt electrohydrodynamic printing process. Macromol Mater Eng, 307: 2100766. https://doi.org/10.1002/mame.202100766
31. Nguyen NT, Kim JH, Jeong YH, 2019, Identification of sagging in melt-electrospinning of microfiber scaffolds. Mater Sci Eng C, 103: 109785. https://doi.org/10.1016/j.msec.2019.109785
32. Zhang F, Cao K, Zaeri A, et al., 2022, Effects of printing sequence on the printing accuracy of melt electrowriting scaffolds. Macromol Mater Eng, 307: 2200222. https://doi.org/10.1002/mame.202200222
33. Cao K, Zhang F, Zaeri A, et al., 2021, A charge-based mechanistic study into the effect of collector temperature on melt electrohydrodynamic printing outcomes. Adv Mater Technol, 6: 2100251. https://doi.org/10.1002/admt.202100251
34. Cao K, Zhang F, Chang RC, 2020, A charge-based mechanistic study into the effects of process parameters on fiber accumulating geometry for a melt electrohydrodynamic process. Processes, 8: 1440. https://doi.org/10.3390/pr8111440
35. Terada A, Okuyama K, Nishikawa M, et al., 2012, The effect of surface charge property on Escherichia coli initial adhesion and subsequent biofilm formation. Biotechnol Bioeng, 109: 1745–1754. https://doi.org/10.1002/bit.24429