Biomaterial inks for extrusion-based 3D bioprinting: Property, classification, modification, and selection
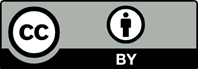
Three-dimensional (3D) extrusion-based bioprinting is the most widely used bioprinting technology to fabricate bionic tissue or organ constructs by combining biomaterial ink and living cells for tissue engineering and regenerative medicine. One critical issue of this technique is the selection of suitable biomaterial ink to simulate extracellular matrix (ECM) that provides mechanical support for cells and regulates their physiological activities. Previous studies have demonstrated that it is an enormous challenge to form and maintain reproducible 3D constructs and eventually achieve the balance among biocompatibility, mechanical properties, and printability. This review highlights the properties of extrusion-based biomaterial inks and recent developments as well as details various biomaterial inks classified by their function. Key approaches related to their modification methods according to the functional requirements are also discussed, along with the selection strategies by varying extrusion paths and methods in extrusion-based bioprinting. This systematical review will assist researchers in identifying the most suitable extrusionbased biomaterial inks based on their requirements, as well as in elaborating current challenges and prospects of extrudable biomaterial inks in the field of bioprinting of in vitro tissue models.
1. Zheng F, Fu F, Cheng Y, et al., 2016, Organ-on-a-chip systems: Microengineering to biomimic living systems. Small, 12(17):2253–2282. https://doi.org/10.1002/smll.201503208
2. Zheng F, Xiao Y, Liu H, et al., 2021, Patient‐specific organoid and organ on‐a‐chip: 3D cell‐culture meets 3D printing and numerical simulation. Adv Biol, 5(6):2000024. https://doi.org/10.1002/adbi.202000024
3. Rawal P, Tripathi DM, Ramakrishna S, et al., 2021, Prospects for 3D bioprinting of organoids. Biodesign Manuf, 4(3): 627–640. https://doi.org/10.1007/s42242-020-00124-1
4. Grigoryan B, Paulsen SJ, Corbett DC, et al., 2019, Multivascular networks and functional intravascular topologies within biocompatible hydrogels. Science, 364(6439):458–464. https://doi.org/10.1126/science.aav9750
5. Lee A, Hudson AR, Shiwarski DJ, et al., 2019, 3D bioprinting of collagen to rebuild components of the human heart. Science, 365(6452):482–487. https://doi.org/10.1126/science.aav9051
6. Groll J, Burdick JA, Cho D-W, et al., 2019, A definition of bioinks and their distinction from biomaterial inks. Biofabrication, 11(1):013001. https://doi.org/10.1088/1758-5090/aaec52
7. Das S, Kim S-W, Choi Y-J, et al., 2019, Decellularized extracellular matrix bioinks and the external stimuli to enhance cardiac tissue development in vitro. Acta Biomater, 95:188–200. https://doi.org/10.1016/j.actbio.2019.04.026
8. Park JY, Ryu H, Lee B, et al., 2019, Development of a functional airway-on-a-chip by 3D cell printing. Biofabrication, 11(1):015002. https://doi.org/10.1088/1758-5090/aae545
9. Kolesky DB, Homan KA, Skylar-Scott MA, et al., 2016, Three-dimensional bioprinting of thick vascularized tissues. Proc Natl Acad Sci USA, 113(12):3179–3184. https://doi.org/10.1073/pnas.1521342113
10. Murphy SV, Atala A, 2014, 3D bioprinting of tissues and organs. Nat Biotechnol, 32(8):773–785. https://doi.org/10.1038/nbt.2958
11. Jungst T, Smolan W, Schacht K, et al., 2016, Strategies and molecular design criteria for 3D printable hydrogels. Chem Rev, 116(3):1496–1539. https://doi.org/10.1021/acs.chemrev.5b00303
12. Bedell ML, Navara AM, Du Y, et al., 2020, Polymeric systems for bioprinting. Chem Rev, 120(19):10744–10792. https://doi.org/10.1021/acs.chemrev.9b00834
13. Hospodiuk M, Dey M, Sosnoski D, et al., 2017, The bioink: A comprehensive review on bioprintable materials. Biotechnol Adv, 35(2):217–239. https://doi.org/10.1016/j.biotechadv.2016.12.006
14. Pati F, Jang J, Lee JW, et al., 2015, Extrusion bioprinting, in Essentials of 3D Biofabrication and Translation, Elsevier, 123–152.
15. Zhang YS, Haghiashtiani G, Hübscher T, et al., 2021, 3D extrusion bioprinting. Nat Rev Methods Primers, 1(1):75. https://doi.org/10.1038/s43586-021-00073-8
16. Ozbolat IT, Hospodiuk M, 2016, Current advances and future perspectives in extrusion-based bioprinting. Biomaterials, 76:321–343. https://doi.org/10.1016/j.biomaterials.2015.10.076
17. Askari M, Afzali Naniz M, Kouhi M, et al., 2021, Recent progress in extrusion 3D bioprinting of hydrogel biomaterials for tissue regeneration: A comprehensive review with focus on advanced fabrication techniques. Biomater Sci, 9(3): 535–573. https://doi.org/10.1039/d0bm00973c
18. Panwar A, Tan LP, 2016, Current status of bioinks for micro extrusion- based 3D bioprinting. Molecules, 21(6):685. https://doi.org/10.3390/molecules21060685
19. Tarassoli SP, Jessop ZM, Jovic T, et al., 2021, Candidate bioinks for extrusion 3D bioprinting—A systematic review of the literature. Front Bioeng Biotech, 9:616753. https://doi.org/10.3389/fbioe.2021.616753
20. Ojansivu M, Rashad A, Ahlinder A, et al., 2019, Wood-based nanocellulose and bioactive glass modified gelatin-alginate bioinks for 3D bioprinting of bone cells. Biofabrication, 11(3):035010. https://doi.org/10.1088/1758-5090/ab0692
21. Zeng X, Meng Z, He J, et al., 2022, Embedded bioprinting for designer 3D tissue constructs with complex structural organization. Acta Biomater, 140:1–22. https://doi.org/10.1016/j.actbio.2021.11.048
22. Colosi C, Shin SR, Manoharan V, et al., 2016, Microfluidic bioprinting of heterogeneous 3D tissue constructs using low‐viscosity bioink. Adv Mater, 28(4):677–684. https://doi.org/10.1002/adma.201503310
23. Ahn G, Min K-H, Kim C, et al., 2017, Precise stacking of decellularized extracellular matrix based 3D cell-laden constructs by a 3D cell printing system equipped with heating modules. Sci Rep-Uk, 7(1):8624. https://doi.org/10.1038/s41598-017-09201-5
24. Ouyang L, Highley CB, Sun W, et al., 2017, A generalizable strategy for the 3D bioprinting of hydrogels from nonviscous photo-crosslinkable inks. Adv Mater, 29(8):1604983. https://doi.org/10.1002/adma.201604983
25. Gao Q, He Y, Fu J-z, et al., 2015, Coaxial nozzle-assisted 3D bioprinting with built-in microchannels for nutrients delivery. Biomaterials, 61:203–215. https://doi.org/10.1016/j.biomaterials.2015.05.031
26. Jin Y, Liu C, Chai W, et al., 2017, Self-supporting nanoclay as internal scaffold material for direct printing of soft hydrogel composite structures in air. ACS Appl Mater Interfaces, 9(20):17456–17465. https://doi.org/10.1021/acsami.7b03613
27. Pi Q, Maharjan S, Yan X, et al., 2018, Digitally tunable microfluidic bioprinting of multilayered cannular tissues. Adv Mater, 30(43):1706913. https://doi.org/10.1002/adma.201706913
28. Zheng Z, Eglin D, Alini M, et al., 2021, Visible light-induced 3D bioprinting technologies and corresponding bioink materials for tissue engineering: A review. Engineering, 7(7):966–978. https://doi.org/10.1016/j.eng.2020.05.021
29. Tabriz AG, Hermida MA, Leslie NR, et al., 2015, Three dimensional bioprinting of complex cell laden alginate hydrogel structures. Biofabrication, 7(4):045012. https://doi.org/10.1088/1758-5090/7/4/045012
30. Flores-Torres S, Peza-Chavez O, Kuasne H, et al., 2021, Alginate-gelatin-Matrigel hydrogels enable the development and multigenerational passaging of patient-derived 3D bioprinted cancer spheroid models. Biofabrication, 13(2):025001. https://doi.org/10.1088/1758-5090/abdb87
31. Parak A, Pradeep P, du Toit LC, et al., 2019, Functionalizing bioinks for 3D bioprinting applications. Drug Discov Today, 24(1):198–205. https://doi.org/10.1016/j.drudis.2018.09.012
32. Snyder JE, Hamid Q, Wang C, et al., 2011, Bioprinting cell laden matrigel for radioprotection study of liver by pro-drug conversion in a dual-tissue microfluidic chip. Biofabrication, 3(3):034112. https://doi.org/10.1088/1758-5082/3/3/034112
33. Fedorovich NE, Wijnberg HM, Dhert WJA, et al., 2011, Distinct tissue formation by heterogeneous printing of osteo and endothelial progenitor cells. Tissue Eng A, 17(15-16): 2113–2121. https://doi.org/10.1089/ten.TEA.2011.0019
34. Jorgensen AM, Chou Z, Gillispie G, et al., 2020, Decellularized skin extracellular matrix (dsECM) improves the physical and biological properties of fibrinogen hydrogel for skin bioprinting applications. Nanomaterials Basel, 10(8):1484. https://doi.org/10.3390/nano10081484
35. Toprakhisar B, Nadernezhad A, Bakirci E, et al., 2018, Development of bioink from decellularized tendon extracellular matrix for 3D bioprinting. Macromol Biosci, 18(10):e1800024. https://doi.org/10.1002/mabi.201800024
36. Hiller T, Berg J, Elomaa L, et al., 2018, Generation of a 3D liver model comprising human extracellular matrix in an alginate/gelatin-based bioink by extrusion bioprinting for infection and transduction studies. Int J Mol Sci, 19(10):3129. https://doi.org/10.3390/ijms19103129
37. Skardal A, Devarasetty M, Kang H-W, et al., 2015, A hydrogel bioink toolkit for mimicking native tissue biochemical and mechanical properties in bioprinted tissue constructs. Acta Biomater, 25:24–34. https://doi.org/10.1016/j.actbio.2015.07.030
38. Visscher DO, Lee H, van Zuijlen PPM, et al., 2021, A photocross linkable cartilage-derived extracellular matrix bioink for auricular cartilage tissue engineering. Acta Biomater, 121:193–203. https://doi.org/10.1016/j.actbio.2020.11.029
39. Gao G, Lee JH, Jang J, et al., 2017, Tissue engineered bio blood-vessels constructed using a tissue-specific bioink and 3D coaxial cell printing technique: A novel therapy for ischemic disease. Adv Funct Mater, 27(33):1700798. https://doi.org/10.1002/adfm.201700798
40. Campos DFD, Blaeser A, Korsten A, et al., 2015, The stiffness and structure of three-dimensional printed hydrogels direct the differentiation of mesenchymal stromal cells toward adipogenic and osteogenic lineages. Tissue Eng A, 21(3-4): 740–756. https://doi.org/10.1089/ten.tea.2014.0231
41. Moncal KK, Ozbolat V, Datta P, et al., 2019, Thermally controlled extrusion-based bioprinting of collagen. J Mater Sci Mater M, 30(5):55. https://doi.org/10.1007/s10856-019-6258-2
42. Skardal A, Mack D, Kapetanovic E, et al., 2012, Bioprinted amniotic fluid-derived stem cells accelerate healing of large skin wounds. Stem Cell Transl Med, 1(11):792–802. https://doi.org/10.5966/sctm.2012-0088
43. Smith CM, Stone AL, Parkhill RL, et al., 2004, Three dimensional bioassembly tool for generating viable tissue engineered constructs. Tissue Eng, 10(9-10):1566–1576. https://doi.org/10.1089/ten.2004.10.1566
44. Poldervaart MT, Goversen B, De Ruijter M, et al., 2017, 3D bioprinting of methacrylated hyaluronic acid (MeHA) hydrogel with intrinsic osteogenicity. PLoS One, 12(6):e0177628. https://doi.org/10.1371/journal.pone.0177628
45. Antich C, de Vicente J, Jiménez G, et al., 2020, Bio-inspired hydrogel composed of hyaluronic acid and alginate as a potential bioink for 3D bioprinting of articular cartilage engineering constructs. Acta Biomater, 106:114–123. https://doi.org/10.1016/j.actbio.2020.01.046
46. Jorgensen AM, Varkey M, Gorkun A, et al., 2020, Bioprinted skin recapitulates normal collagen remodeling in full thickness wounds. Tissue Eng A, 26(9-10):512–526. https://doi.org/10.1089/ten.TEA.2019.0319
47. Law N, Doney B, Glover H, et al., 2018, Characterisation of hyaluronic acid methylcellulose hydrogels for 3D bioprinting. J Mech Behav Biomed, 77:389–399. https://doi.org/10.1016/j.jmbbm.2017.09.031
48. Kiyotake EA, Douglas AW, Thomas EE, et al., 2019, Development and quantitative characterization of the precursor rheology of hyaluronic acid hydrogels for bioprinting. Acta Biomater, 95:176–187. https://doi.org/10.1016/j.actbio.2019.01.041
49. Raddatz L, Lavrentieva A, Pepelanova I, et al., 2018, Development and application of an additively manufactured calcium chloride Nebulizer for alginate 3D-bioprinting purposes. J Funct Biomat, 9(4):63. https://doi.org/10.3390/jfb9040063
50. Kim MH, Lee YW, Jung W-K, et al., 2019, Enhanced rheological behaviors of alginate hydrogels with carrageenan for extrusion-based bioprinting. J Mech Behav Biomed, 98:187–194. https://doi.org/10.1016/j.jmbbm.2019.06.014
51. Ooi HW, Mota C, Ten Cate AT, et al., 2018, Thiol-ene alginate hydrogels as versatile bioinks for bioprinting. Biomacromolecules, 19(8):3390–3400. https://doi.org/10.1021/acs.biomac.8b00696
52. Markstedt K, Mantas A, Tournier I, et al., 2015, 3D bioprinting human chondrocytes with nano cellulose alginate bioink for cartilage tissue engineering applications. Biomacromolecules, 16(5):1489–1496. https://doi.org/10.1021/acs.biomac.5b00188
53. Khalil S, Sun W, 2009, Bioprinting endothelial cells with alginate for 3D tissue constructs. J Biomech Eng-T Asme, 131(11):111002. https://doi.org/10.1115/1.3128729
54. Hafezi F, Shorter S, Tabriz AG, et al., 2020, Bioprinting and preliminary testing of highly reproducible novel bioink for potential skin regeneration. Pharmaceutics, 12(6):550. https://doi.org/10.3390/pharmaceutics12060550
55. Maturavongsadit P, Narayanan LK, Chansoria P, et al., 2021, Cell-laden nanocellulose/chitosan-based bioinks for 3D bioprinting and enhanced osteogenic cell differentiation. ACS Appl Bio Mater, 4(3):2342–2353. https://doi.org/10.1021/acsabm.0c01108
56. Ku J, Seonwoo H, Park S, et al., 2020, Cell-laden thermosensitive chitosan hydrogel bioinks for 3D bioprinting applications. Appl Sci Basel, 10(7):2455. https://doi.org/10.3390/app10072455
57. Ng WL, Yeong WY, Naing MW, 2016, Polyelectrolyte gelatin-chitosan hydrogel optimized for 3D bioprinting in skin tissue engineering. Int J Bioprint, 2(1):53–62. https://doi.org/10.18063/IJB.2016.01.009
58. Liu Q, Li Q, Xu S, et al., 2018, Preparation and properties of 3D printed alginate-chitosan polyion complex hydrogels for tissue engineering. Polymers Basel, 10(6):664. https://doi.org/10.3390/polym10060664
59. Wu Q, Therriault D, Heuzey M-C, 2018, Processing and properties of chitosan inks for 3D printing of hydrogel microstructures. ACS Biomater Sci Eng, 4(7):2643–2652. https://doi.org/10.1021/acsbiomaterials.8b00415
60. Bergonzi C, Di Natale A, Zimetti F, et al., 2019, Study of 3D-printed chitosan scaffold features after different post printing gelation processes. Sci Rep UK, 9(1):362. https://doi.org/10.1038/s41598-018-36613-8
61. Sharma R, Smits IPM, De La Vega L, et al., 2020, 3D bioprinting pluripotent stem cell derived neural tissues using a novel fibrin bioink containing drug releasing microspheres. Front Bioeng Biotech, 8:57. https://doi.org/10.3389/fbioe.2020.00057
62. England S, Rajaram A, Schreyer DJ, et al., 2017, Bioprinted fibrin-factor XIII-hyaluronate hydrogel scaffolds with encapsulated Schwann cells and their in vitro characterization for use in nerve regeneration. Bioprinting, 5:1–9. https://doi.org/10.1016/j.bprint.2016.12.001
63. Lee C, Abelseth E, de la Vega L, et al., 2019, Bioprinting a novel glioblastoma tumor model using a fibrin-based bioink for drug screening. Mater Today Chem, 12:78–84. https://doi.org/10.1016/j.mtchem.2018.12.005
64. Ning L, Zhu N, Mohabatpour F, et al., 2019, Bioprinting Schwann cell-laden scaffolds from low-viscosity hydrogel compositions. J Mater Chem B, 7(29):4538–4551. https://doi.org/10.1039/c9tb00669a
65. Anil Kumar S, Alonzo M, Allen SC, et al., 2019, A visible light-cross-linkable, fibrin-gelatin-based bioprinted construct with human cardiomyocytes and fibroblasts. ACS Biomater Sci Eng, 5(9):4551–4563. https://doi.org/10.1021/acsbiomaterials.9b00505
66. Rodriguez MJ, Dixon TA, Cohen E, et al., 2018, 3D freeform printing of silk fibroin. Acta Biomater, 71:379–387. https://doi.org/10.1016/j.actbio.2018.02.035
67. Ni T, Liu M, Zhang Y, et al., 2020, 3D bioprinting of bone marrow mesenchymal stem cell-laden silk fibroin double network scaffolds for cartilage tissue repair. Bioconjug Chem, 31(8):1938–1947. https://doi.org/10.1021/acs.bioconjchem.0c00298
68. Zheng Z, Wu J, Liu M, et al., 2018, 3D bioprinting of self standing silk-based bioink. Adv Healthc Mater, 7(6):1701026. https://doi.org/10.1002/adhm.201701026
69. Wu D, Yu Y, Tan J, et al., 2018, 3D bioprinting of gellan gum and poly (ethylene glycol) diacrylate based hydrogels to
produce human-scale constructs with high-fidelity. Mater Design, 160:486–495. https://doi.org/10.1016/j.matdes.2018.09.040
70. Chen Y, Xiong X, Liu X, et al., 2020, 3D bioprinting of shear thinning hybrid bioinks with excellent bioactivity derived from gellan/alginate and thixotropic magnesium phosphate based gels. J Mater Chem B, 8(25):5500–5514. https://doi.org/10.1039/d0tb00060d
71. Zhu S, Yao L, Pan C, et al., 2021, 3D printed gellan gum/graphene oxide scaffold for tumor therapy and bone reconstruction. Compos Sci Technol, 208:108763. https://doi.org/10.1016/j.compscitech.2021.108763
72. Lozano R, Stevens L, Thompson BC, et al., 2015, 3D printing of layered brain-like structures using peptide modified gellan gum substrates. Biomaterials, 67:264-273. https://doi.org/10.1016/j.biomaterials.2015.07.022
73. Mouser VHM, Melchels FPW, Visser J, et al., 2016, Yield stress determines bioprintability of hydrogels based on gelatin-methacryloyl and gellan gum for cartilage bioprinting. Biofabrication, 8(3):035003. https://doi.org/10.1088/1758-5090/8/3/035003
74. Cleymand F, Poerio A, Mamanov A, et al., 2021, Development of novel chitosan/guar gum inks for extrusion-based 3D bioprinting: Process, printability and properties. Bioprinting, 21:e00122. https://doi.org/10.1016/j.bprint.2020.e00122
75. Indurkar A, Bangde P, Gore M, et al., 2020, Optimization of guar gum-gelatin bioink for 3D printing of mammalian cells. Bioprinting, 20:e00101. https://doi.org/10.1016/j.bprint.2020.e00101
76. Muthulakshmi L, Pavithra U, Sivaranjani V, et al., 2021, A novel Ag/carrageenan–gelatin hybrid hydrogel nanocomposite and its biological applications: Preparation and characterization. J Mech Behav Biomed, 115:104257. https://doi.org/10.1016/j.jmbbm.2020.104257
77. Li H, Tan YJ, Liu S, et al., 2018, Three-dimensional bioprinting of oppositely charged hydrogels with super strong interface bonding. ACS Appl Mater Interfaces, 10(13):11164–11174. https://doi.org/10.1021/acsami.7b19730
78. Lim W, Kim GJ, Kim HW, et al., 2020, Kappa-carrageenan based dual cross linkable bioink for extrusion type bioprinting. Polymers Basel, 12(10):2377. https://doi.org/10.3390/polym12102377
79. Gong C, Kong Z, Wang X, 2021, The effect of agarose on 3D bioprinting. Polymers Basel, 13(22):4028. https://doi.org/10.3390/polym13224028
80. Tan YJ, Tan X, Yeong WY, et al., 2016, Hybrid microscaffold based 3D bioprinting of multi-cellular constructs with high compressive strength: A new biofabrication strategy. Sci Rep UK, 6(1):39140. https://doi.org/10.1038/srep39140
81. Massa S, Sakr MA, Seo J, et al., 2017, Bioprinted 3D vascularized tissue model for drug toxicity analysis. Biomicrofluidics, 11(4):044109. https://doi.org/10.1063/1.4994708
82. Singh YP, Bandyopadhyay A, Mandal BB, 2019, 3D bioprinting using cross-linker-free silk–gelatin bioink for cartilage tissue engineering. ACS Appl Mater Interfaces, 11(37):33684–33696. https://doi.org/10.1021/acsami.9b11644
83. Schwartz R, Malpica M, Thompson GL, et al., 2020, Cell encapsulation in gelatin bioink impairs 3D bioprinting resolution. J Mech Behav Biomed, 103:103524. https://doi.org/10.1016/j.jmbbm.2019.103524
84. Zehnder T, Sarker B, Boccaccini AR, et al., 2015, Evaluation of an alginate–gelatine crosslinked hydrogel for bioplotting. Biofabrication, 7(2):025001. https://doi.org/10.1088/1758-5090/7/2/025001
85. Song K, Compaan AM, Chai W, et al., 2020, Injectable gelatin microgel-based composite ink for 3D bioprinting in air. ACS Appl Mater Interfaces, 12(20):22453–22466. https://doi.org/10.1021/acsami.0c01497
86. Leucht A, Volz A-C, Rogal J, et al., 2020, Advanced gelatin-based vascularization bioinks for extrusion-based bioprinting of vascularized bone equivalents. Sci Rep UK, 10(1):5330. https://doi.org/10.1038/s41598-020-62166-w
87. Kolan KCR, Semon JA, Bromet B, et al., 2019, Bioprinting with human stem cell-laden alginate-gelatin bioink and bioactive glass for tissue engineering. Int J Bioprint, 5(2.2):204. https://doi.org/10.18063/ijb.v5i2.2.204
88. Yin J, Yan M, Wang Y, et al., 2020, In vitro and in vivo biocompatibility evaluation of a 3D bioprinted gelatin sodium alginate/rat Schwann-cell scaffold. Mater Sci Eng C Mater, 109:110530. https://doi.org/10.1016/j.msec.2019.110530
89. Yin J, Yan ML, Wang YC, et al., 2018, 3D bioprinting of low-concentration cell-laden gelatin methacrylate (GelMA) bioinks with a two-step cross-linking strategy. ACS Appl Mater Interfaces, 10(8):6849–6857. https://doi.org/10.1021/acsami.7b16059
90. Rastin H, Ormsby RT, Atkins GJ, et al., 2020, 3D bioprinting of methylcellulose/gelatin-methacryloyl (MC/GelMA) bioink with high shape integrity. ACS Appl Biomater, 3(3):1815–1826. https://doi.org/10.1021/acsabm.0c00169
91. Erdem A, Darabi MA, Nasiri R, et al., 2020, 3D bioprinting of oxygenated cell‐laden gelatin methacryloyl constructs. Adv Healthc Mater, 9(15):1901794. https://doi.org/10.1002/adhm.201901794
92. Colle J, Blondeel P, De Bruyne A, et al., 2020, Bioprinting pre-differentiated adipose-derived mesenchymal stem cell spheroids with methacrylated gelatin ink for adipose tissue engineering. J Mater Sci Mater M, 31(4):36. https://doi.org/10.1007/s10856-020-06374-w
93. Ning L, Mehta R, Cao C, et al., 2020, Embedded 3D bioprinting of gelatin methacryloyl-based constructs with highly tunable structural fidelity. ACS Appl Mater Interfaces, 12(40):44563–44577. https://doi.org/10.1021/acsami.0c15078
94. Liu W, Heinrich MA, Zhou Y, et al., 2017, Extrusion bioprinting of shear‐thinning gelatin methacryloyl bioinks. Adv Healthc Mater, 6(12):1601451. https://doi.org/10.1002/adhm.201601451
95. Wu Y, Wenger A, Golzar H, et al., 2020, 3D bioprinting of bicellular liver lobule-mimetic structures via microextrusion of cellulose nanocrystal-incorporated shear-thinning bioink. Sci Rep UK, 10(1):20648. https://doi.org/10.1038/s41598-020-77146-3
96. Li H, Tan YJ, Leong KF, et al., 2017, 3D bioprinting of highly thixotropic alginate/methylcellulose hydrogel with strong interface bonding. ACS Appl Mater & Interfaces, 9(23):20086–20097. https://doi.org/10.1021/acsami.7b04216
97. Frost BA, Sutliff BP, Thayer P, et al., 2019, Gradient poly(ethylene glycol) diacrylate and cellulose nanocrystals tissue engineering composite scaffolds via extrusion bioprinting. Front Bioeng Biotech, 7:280. https://doi.org/10.3389/fbioe.2019.00280
98. Li Z, Ramos A, Li M-C, et al., 2020, Improvement of cell deposition by self-absorbent capability of freeze-dried 3D-bioprinted scaffolds derived from cellulose material alginate hydrogels. Biomed Phys Eng Express, 6(4): 045009. https://doi.org/10.1088/2057-1976/ab8fc6
99. Ji S, Abaci A, Morrison T, et al., 2020, Novel bioinks from UV-responsive norbornene-functionalized carboxymethyl cellulose macromers. Bioprinting, 18:e00083. https://doi.org/10.1016/j.bprint.2020.e00083
100. Wu Y, Heikal L, Ferns G, et al., 2019, 3D bioprinting of novel biocompatible scaffolds for endothelial cell repair. Polymers Basel, 11(12):1924. https://doi.org/10.3390/polym11121924
101. Xin S, Chimene D, Garza JE, et al., 2019, Clickable PEG hydrogel microspheres as building blocks for 3D bioprinting. Biomater Sci, 7(3):1179–1187. https://doi.org/10.1039/C8BM01286E
102. Hong S, Kim JS, Jung B, et al., 2019, Coaxial bioprinting of cell-laden vascular constructs using a gelatin–tyramine bioink. Biomater Sci, 7(11):4578–4587. https://doi.org/10.1039/C8BM00618K
103. Sun X, Ma Z, Zhao X, et al., 2021, Three-dimensional bioprinting of multicell-laden scaffolds containing bone morphogenic protein-4 for promoting M2 macrophage polarization and accelerating bone defect repair in diabetes mellitus. Bioactive Mater, 6(3):757–769. https://doi.org/10.1016/j.bioactmat.2020.08.030
104. Hu D, Wu D, Huang L, et al., 2018, 3D bioprinting of cell laden scaffolds for intervertebral disc regeneration. Mater Lett, 223:219–222. https://doi.org/10.1016/j.matlet.2018.03.204
105. Shin YJ, Shafranek RT, Tsui JH, et al., 2021, 3D bioprinting of mechanically tuned bioinks derived from cardiac decellularized extracellular matrix. Acta Biomater, 119:75–88. https://doi.org/10.1016/j.actbio.2020.11.006
106. Kolesky DB, Truby RL, Gladman AS, et al., 2014, 3D bioprinting of vascularized, heterogeneous cell-laden tissue constructs. Adv Mater, 26(19):3124–3130. https://doi.org/10.1002/adma.201305506
107. Gori M, Giannitelli SM, Torre M, et al., 2020, Biofabrication of hepatic constructs by 3D bioprinting of a cell-laden thermogel: An effective tool to assess drug-induced hepatotoxic response. Adv Healthc Mater, 9(21):e2001163. https://doi.org/10.1002/adhm.202001163
108. Suntornnond R, Tan EYS, An J, et al., 2017, A highly printable and biocompatible hydrogel composite for direct printing of soft and perfusable vasculature-like structures. Sci Rep UK, 7(1):16902. https://doi.org/10.1038/s41598-017-17198-0
109. Suntornnond R, Tan EYS, An J, et al., 2016, A mathematical model on the resolution of extrusion bioprinting for the development of new bioinks. Materials, 9(9):756. https://doi.org/10.3390/ma9090756
110. Lee J, Kim G, 2018, Three-dimensional hierarchical nanofibrous collagen scaffold fabricated using fibrillated collagen and Pluronic F-127 for regenerating bone tissue. ACS Appl Mater Interfaces, 10(42):35801–35811. https://doi.org/10.1021/acsami.8b14088
111. Kundu J, Shim J-H, Jang J, et al., 2015, An additive manufacturing-based PCL–alginate–chondrocyte bioprinted scaffold for cartilage tissue engineering. J Tissue Eng Regen Med, 9(11):1286–1297. https://doi.org/10.1002/term.1682
112. Vijayavenkataraman S, Vialli N, Fuh JYH, et al., 2019, Conductive collagen/polypyrrole-b-polycaprolactone hydrogel for bioprinting of neural tissue constructs. Int J Bioprint, 5(2.1):31–43. https://doi.org/10.18063/ijb.v5i2.1.229
113. Kim BS, Jang J, Chae S, et al., 2016, Three-dimensional bioprinting of cell-laden constructs with polycaprolactone protective layers for using various thermoplastic polymers. Biofabrication, 8(3):035013. https://doi.org/10.1088/1758-5090/8/3/035013
114. Narayanan LK, Huebner P, Fisher MB, et al., 2016, 3D-bioprinting of polylactic acid (PLA) nanofiber–alginate hydrogel bioink containing human adipose-derived stem cells. ACS Biomater Sci Eng, 2(10):1732–1742. https://doi.org/10.1021/acsbiomaterials.6b00196
115. Kolan KCR, Semon JA, Bindbeutel AT, et al., 2020, Bioprinting with bioactive glass loaded polylactic acid composite and human adipose stem cells. Bioprinting, 18:e00075. https://doi.org/10.1016/j.bprint.2020.e00075
116. Aydogdu MO, Oner ET, Ekren N, et al., 2019, Comparative characterization of the hydrogel added PLA/β-TCP scaffolds produced by 3D bioprinting. Bioprinting, 13:e00046. https://doi.org/10.1016/j.bprint.2019.e00046
117. Zamani Y, Mohammadi J, Amoabediny G, et al., 2020, Bioprinting of alginate-encapsulated pre-osteoblasts in PLGA/β-TCP scaffolds enhances cell retention but impairs osteogenic differentiation compared to cell seeding after 3D-printing. Regen Eng Transl Med, 7(4):485–493. https://doi.org/10.1007/s40883-020-00163-1
118. Naseri E, Butler H, Macnevin W, et al., 2020, Low-temperature solvent-based 3D printing of PLGA: A parametric printability study. Drug Dev Ind Pharm, 46(2):173–178. https://doi.org/10.1080/03639045.2019.1711389
119. Huang J, Huang Z, Liang Y, et al., 2021, 3D printed gelatin/hydroxyapatite scaffolds for stem cell chondrogenic differentiation and articular cartilage repair. Biomater Sci, 9(7):2620–2630. https://doi.org/10.1039/D0BM02103B
120. Wenz A, Borchers K, Tovar GEM, et al., 2017, Bone matrix production in hydroxyapatite-modified hydrogels suitable for bone bioprinting. Biofabrication, 9(4):044103. https://doi.org/10.1088/1758-5090/aa91ec
121. Bendtsen ST, Quinnell SP, Wei M, 2017, Development of a novel alginate-polyvinyl alcohol-hydroxyapatite hydrogel for 3D bioprinting bone tissue engineered scaffolds. J Biomed Mater Res A, 105(5):1457–1468. https://doi.org/10.1002/jbm.a.36036
122. Adhikari J, Perwez MS, Das A, et al., 2021, Development of hydroxyapatite reinforced alginate–chitosan based printable biomaterial-ink. Nanostruct Nanoobjects, 25:100630. https://doi.org/10.1016/j.nanoso.2020.100630
123. Lin K-F, He S, Song Y, et al., 2016, Low-temperature additive manufacturing of biomimic three-dimensional hydroxyapatite/collagen scaffolds for bone regeneration. ACS Appl Mater Interfaces, 8(11):6905–6916. https://doi.org/10.1021/acsami.6b00815
124. Gao Q, Niu X, Shao L, et al., 2019, 3D printing of complex GelMA-based scaffolds with nanoclay. Biofabrication, 11(3):035006. https://doi.org/10.1088/1758-5090/ab0cf6
125. Hu C, Hahn L, Yang M, et al., 2021, Improving printability of a thermoresponsive hydrogel biomaterial ink by nanoclay addition. J Mater Sci, 56(1):691–705. https://doi.org/10.1007/s10853-020-05190-5
126. Cidonio 1 G, Glinka M, Kim Y-H, et al., 2020, Nanoclay based 3D printed scaffolds promote vascular ingrowth ex vivo and generate bone mineral tissue in vitro and in vivo. Biofabrication, 12(3):035010. https://doi.org/10.1088/1758-5090/ab8753
127. Zhu W, Cui H, Boualam B, et al., 2018, 3D bioprinting mesenchymal stem cell-laden construct with core-shell nanospheres for cartilage tissue engineering. Nanotechnology, 29(18): 185101. https://doi.org/10.1088/1361-6528/aaafa1
128. Swaminathan S, Hamid Q, Sun W, et al., 2019, Bioprinting of 3D breast epithelial spheroids for human cancer models. Biofabrication, 11(2):025003. https://doi.org/10.1088/1758-5090/aafc49
129. Carrow JK, Kerativitayanan P, Jaiswal MK, et al., 2015, Polymers for bioprinting, in Essentials of 3D Biofabrication and Translation, Atala A and Yoo JJ, Academic Press, Boston, 229–248.
130. Nijenhuis KT, 1997, Thermoreversible Networks: Viscoelastic Properties and Structure of Gels, 1 edn, Springer, Berlin, Heidelberg.
131. Lee VK, Lanzi AM, Ngo H, et al., 2014, Generation of multiscale vascular network system within 3D hydrogel using 3D bio-printing technology. Cell Mol Bioeng, 7(3):460–472. https://doi.org/10.1007/s12195-014-0340-0.
132. Zhao L, Lee VK, Yoo S-S, et al., 2012, The integration of 3-D cell printing and mesoscopic fluorescence molecular tomography of vascular constructs within thick hydrogel scaffolds. Biomaterials, 33(21):5325–5332. https://doi.org/10.1016/j.biomaterials.2012.04.004
133. Sun Y, Yu K, Nie J, et al., 2021, Modeling the printability of photocuring and strength adjustable hydrogel bioink during projection-based 3D bioprinting. Biofabrication, 13(3):035032. https://doi.org/10.1088/1758-5090/aba413
134. Iliyana P, Katharina K, Thomas S, et al., 2018, Gelatin methacryloyl (GelMA) hydrogels with defined degree of functionalization as a versatile toolkit for 3D cell culture and extrusion bioprinting. Bioengineering, 5(3):55. https://doi.org/10.3390/bioengineering5030055
135. Lee BH, Lum N, Seow LY, et al., 2016, Synthesis and characterization of types A and B gelatin methacryloyl for bioink applications. Materials, 9(10):797. https://doi.org/10.3390/ma9100797
136. Cubo N, Garcia M, CaÃizo JFD, et al., 2017, 3D bioprinting of functional human skin: Production and in vivo analysis. Biofabrication, 9(11):2843–2854. https://doi.org/10.1088/1758-5090/9/1/015006
137. Ouyang L, Yao R, Chen X, et al., 2015, 3D printing of HEK 293FT cell-laden hydrogel into macroporous constructs with high cell viability and normal biological functions. Biofabrication, 7(1): 015010. https://doi.org/10.1088/1758-5090/7/1/015010
138. Testa S, Mozetic P, Barbetta A, et al., 2017, Microfluidic enhanced 3D bioprinting of aligned myoblast-laden hydrogels leads to functionally organized myofibers in vitro and in vivo. Biomaterials, 131:98–110. https://doi.org/10.1016/j.biomaterials.2017.03.026
139. Pescosolido L, Schuurman W, Malda J, et al., 2011, Hyaluronic acid and dextran-based semi-IPN hydrogels as biomaterials for bioprinting. Biomacromolecules, 12(5):1831–1838. https://doi.org/10.1021/bm200178w
140. Park JY, Choi J-C, Shim J-H, et al., 2014, A comparative study on collagen type I and hyaluronic acid dependent cell behavior for osteochondral tissue bioprinting. Biofabrication, 6(3):035004. https://doi.org/10.1088/1758-5082/6/3/035004
141. Ma L, Li Y, Wu Y, et al., 2020, 3D bioprinted hyaluronic acid-based cell-laden scaffold for brain microenvironment simulation. Biodesign Manuf, 3(3):164–174. https://doi.org/10.1007/s42242-020-00076-6
142. Wibowo A, Vyas C, Cooper G, et al., 2020, 3D printing of polycaprolactone-polyaniline electroactive scaffolds for bone tissue engineering. Materials, 13(3):512. https://doi.org/10.3390/ma13030512
143. Zhao H, Xu J, Zhang E, et al., 2021, 3D bioprinting of polythiophene materials for promoting stem cell proliferation in a nutritionally deficient environment. ACS Appl Mater Interfaces, 13(22):25759–25770. https://doi.org/10.1021/acsami.1c04967
144. Yuk H, Lu B, Lin S, et al., 2020, 3D printing of conducting polymers. Nat Commun, 11(1):1604. https://doi.org/10.1038/s41467-020-15316-7
145. Zhu K, Shin SR, van Kempen T, et al., 2017, Gold nanocomposite bioink for printing 3D cardiac constructs. Adv Funct Mater, 27(12):1605352. https://doi.org/10.1002/adfm.201605352
146. Mannoor MS, Jiang Z, James T, et al., 2013, 3D printed bionic ears. Nano Lett, 13(6):2634–2639. https://doi.org/10.1021/nl4007744
147. Mehrotra S, Singh RD, Bandyopadhyay A, et al., 2021, Engineering microsphere-loaded non-mulberry silk-based 3D bioprinted vascularized cardiac patches with oxygen releasing and immunomodulatory potential. ACS Appl Mater Interfaces, 13(43):50744–50759. https://doi.org/10.1021/acsami.1c14118
148. Asulin M, Michael I, Shapira A, et al., 2021, One-step 3D printing of heart patches with built-in electronics for performance regulation. Adv Sci, 8(9):2004205. https://doi.org/10.1002/advs.202004205
149. Tian K, Bae J, Bakarich SE, et al., 2017, 3D printing of transparent and conductive heterogeneous hydrogel elastomer systems. Adv Mater, 29(10):1604827. https://doi.org/10.1002/adma.201604827
150. Jia J, Richards DJ, Pollard S, et al., 2014, Engineering alginate as bioink for bioprinting. Acta Biomater, 10(10):4323–4331. https://doi.org/10.1016/j.actbio.2014.06.034
151. Kang D, Hong G, An S, et al., 2020, Bioprinting of multiscaled hepatic lobules within a highly vascularized construct. Small, 16(13):1905505. https://doi.org/10.1002/smll.201905505
152. Yegappan R, Selvaprithiviraj V, Amirthalingam S, et al., 2018, Carrageenan based hydrogels for drug delivery, tissue engineering and wound healing. Carbohydr Polym, 198: 385–400. https://doi.org/10.1016/j.carbpol.2018.06.086
153. Nadernezhad A, Caliskan OS, Topuz F, et al., 2019, Nanocomposite bioinks based on agarose and 2D nanosilicates with tunable flow properties and bioactivity for 3D bioprinting. ACS Appl Biomater, 2(2):796–806. https://doi.org/10.1021/acsabm.8b00665
154. Bertassoni LE, Cecconi M, Manoharan V, et al., 2014, Hydrogel bioprinted microchannel networks for
vascularization of tissue engineering constructs. Lab Chip, 14(13):2202–2211. https://doi.org/10.1039/c4lc00030g
155. Mirdamadi E, Muselimyan N, Koti P, et al., 2019, Agarose slurry as a support medium for bioprinting and culturing freestanding cell-laden hydrogel constructs. 3D Print Addit Manuf, 6(3):158–164. https://doi.org/10.1089/3dp.2018.0175
156. Fedorovich NE, Swennen I, Girones J, et al., 2009, Evaluation of photocross linked lutrol hydrogel for tissue printing applications. Biomacromolecules, 10(7):1689–1696. https://doi.org/10.1021/bm801463q
157. Lewis PL, Yan M, Su J, et al., 2019, Directing the growth and alignment of biliary epithelium within extracellular matrix hydrogels. Acta Biomater, 85:84–93. https://doi.org/10.1016/j.actbio.2018.12.039
158. Veronese FM, Pasut G, 2005, PEGylation, successful approach to drug delivery. Drug Discov Today, 10(21):1451–1458. https://doi.org/10.1016/s1359-6446(05)03575-0
159. Floody MC, Theng BKG, Reyes P, et al., 2009, Natural nanoclays: Applications and future trends—A Chilean perspective. Clay Miner, 44(2):161–176. https://doi.org/10.1180/claymin.2009.044.2.161
160. Xavier JR, Thakur T, Desai P, et al., 2015, Bioactive nanoengineered hydrogels for bone tissue engineering: A growth-factor-free approach. ACS Nano, 9(3):3109–3118. https://doi.org/10.1021/nn507488s
161. Gladman AS, Matsumoto EA, Nuzzo RG, et al., 2016, Biomimetic 4D printing. Nat Mater, 15(4):413–418. https://doi.org/10.1038/nmat4544
162. Liu J, Erol O, Pantula A, et al., 2019, Dual-gel 4D printing of bioinspired tubes. ACS Appl Mater Interfaces, 11(8):8492–8498. https://doi.org/10.1021/acsami.8b17218
163. Ahlfeld T, Cidonio G, Kilian D, et al., 2017, Development of a clay based bioink for 3D cell printing for skeletal application. Biofabrication, 9(3):034103. https://doi.org/10.1088/1758-5090/aa7e96
164. Mulakkal MC, Trask RS, Ting VP, et al., 2018, Responsive cellulose-hydrogel composite ink for 4D printing. Mater Design, 160:108–118. https://doi.org/10.1016/j.matdes.2018.09.009
165. Zhang X, Zhou Y, Liu Y, et al., 2021, Effect of water soluble polymer on the dispersion stability of cellulose nanofibers in water. J Cell Sci Technol, 28(1):26–33. https://doi.org/10.16561/j.cnki.xws.2020.01.07
166. Lille M, Nurmela A, Nordlund E, et al., 2018, Applicability of protein and fiber-rich food materials in extrusion-based 3D printing. J Food Eng, 220:20–27. https://doi.org/10.1016/j.jfoodeng.2017.04.034
167. Wu Y, Lin ZYW, Wenger AC, et al., 2018, 3D bioprinting of liver-mimetic construct with alginate/cellulose nanocrystal hybrid bioink. Bioprinting, 9:1-6. https://doi.org/10.1016/j.bprint.2017.12.001
168. Giachini P, Gupta SS, Wang W, et al., 2020, Additive manufacturing of cellulose-based materials with continuous, multidirectional stiffness gradients. Sci Adv, 6(8):eaay0929. https://doi.org/10.1126/sciadv.aay0929
169. Gospodinova A, Nankov V, Tomov S, et al., 2021, Extrusion bioprinting of hydroxyethylcellulose-based bioink for cervical tumor model. Carbohydr Polym, 260:117793. https://doi.org/10.1016/j.carbpol.2021.117793
170. Li X, Deng Q, Wang S, et al., 2021, Hydroxyethyl cellulose as a rheological additive for tuning the extrusion printability and scaffold properties. 3D Print Addit Manuf, 8(2):87–98. https://doi.org/10.1089/3dp.2020.0167
171. Mudgil D, Barak S, Khatkar BS, 2014, Guar gum: Processing, properties and food applications—A review. J Food Sci Technol Mysore, 51(3):409–418. https://doi.org/10.1007/s13197-011-0522-x
172. Hahn L, Beudert M, Gutmann M, et al., 2021, From thermogelling hydrogels toward functional bioinks: Controlled modification and cytocompatible crosslinking. Macromol Biosci, 21(10):e2100122. https://doi.org/10.1002/mabi.202100122
173. Daly AC, Cunniffe GM, Sathy BN, et al., 2016, 3D bioprinting of developmentally inspired templates for whole bone organ engineering. Adv Healthc Mater, 5(18):2353–2362. https://doi.org/10.1002/adhm.201600182
174. Sarker MD, Naghieh S, McInnes AD, et al., 2019, Biofabrication of peptide-modified alginate scaffolds: Printability, mechanical stability and neurite outgrowth assessments. Bioprinting, 14:e00045. https://doi.org/10.1016/j.bprint.2019.e00045
175. Barrs RW, Jia J, Ward M, et al., 2021, Engineering a chemically defined hydrogel bioink for direct bioprinting of microvasculature. Biomacromolecules, 22(2):275–288. https://doi.org/10.1021/acs.biomac.0c00947
176. Wang Q, Yang X, Wang G, et al., 2020, Osteogenic growth peptide-loaded 3D-printed PCL scaffolds for the promotion of osteogenesis through the ERK pathway. Mater Design, 193:108811. https://doi.org/10.1016/j.matdes.2020.108811
177. Guo JL, Diaz-Gomez L, Xie VY, et al., 2021, Three dimensional printing of click functionalized, peptide patterned scaffolds for osteochondral tissue engineering. Bioprinting, 22:e00136. https://doi.org/10.1016/j.bprint.2021.e00136
178. Wang X, Ao Q, Tian X, et al., 2017, Gelatin-based hydrogels for organ 3D bioprinting. Polymers Basel, 9(9): 401. https://doi.org/10.3390/polym9090401
179. Hauptstein J, Boeck T, Bartolf-Kopp M, et al., 2020, Hyaluronic acid-based bioink composition enabling 3D bioprinting and improving quality of deposited cartilaginous extracellular matrix. Adv Healthc Mater, 9(15):2000737. https://doi.org/10.1002/adhm.202000737
180. Chameettachal S, Sasikumar S, Sethi S, et al., 2019, Tissue/ organ-derived bioink formulation for 3D bioprinting. J 3D Print Med, 3(1):39–54. https://doi.org/10.2217/3dp-2018-0024
181. Cheng Y, Zheng F, Lu J, et al., 2014, Bioinspired multicompartmental microfibers from microfluidics. Adv Mater, 26(30):5184–5190. https://doi.org/10.1002/adma.201400798
182. Rajput S, Deo KA, Mathur T, et al., 2022, 2D nanosilicate for additive manufacturing: Rheological modifier, sacrificial ink and support bath. Bioprinting, 25:e00187. https://doi.org/10.1016/j.bprint.2021.e00187
183. Liu P, Mu Z, Ji M, et al., 2021, Robust carbonated structural color barcodes with ultralow ontology fluorescence as biomimic culture platform. Research, 2021: 9851609. https://doi.org/10.34133/2021/9851609
184. Lee S, Sani ES, Spencer AR, et al., 2020, Human-recombinant elastin- based bioinks for 3D bioprinting of vascularized soft tissues. Adv Mater, 32(45):e2003915. https://doi.org/10.1002/adma.202003915
185. Park B-W, Jung S-H, Das S, et al., 2020, In vivo priming of human mesenchymal stem cells with hepatocyte growth factor-engineered mesenchymal stem cells promotes therapeutic potential for cardiac repair. Sci Adv, 6(13):eaay6994. https://doi.org/10.1126/sciadv.aay6994
186. Kim W, Kim GH, 2020, An intestinal model with a fingerlike villus structure fabricated using a bioprinting process and collagen/SIS-based cell-laden bioink. Theranostics, 10(6):2495–2508. https://doi.org/10.7150/thno.41225
187. Kim W, Kim G, 2018, Intestinal villi model with blood capillaries fabricated using collagen-based bioink and dual-cell-printing process. ACS Appl Mater Interfaces, 10(48):41185–41196. https://doi.org/10.1021/acsami.8b17410
188. Wang Y, Shi W, Kuss M, et al., 2018, 3D bioprinting of breast cancer models for drug resistance study. ACS Biomater Sci Eng, 4(12):4401–4411. https://doi.org/10.1021/acsbiomaterials.8b01277
189. Bhise NS, Manoharan V, Massa S, et al., 2016, A liveron-a-chip platform with bioprinted hepatic spheroids. Biofabrication, 8(1):014101. https://doi.org/10.1088/1758-5090/8/1/014101
190. Nguyen D, Hagg DA, Forsman A, et al., 2017, Cartilage tissue engineering by the 3D bioprinting of iPS cells in a nanocellulose/alginate bioink. Sci Rep UK, 7:658. https://doi.org/10.1038/s41598-017-00690-y
191. Li X, Deng Q, Zhuang T, et al., 2020, 3D bioprinted breast tumor model for structure-activity relationship study. Biodesign Manuf, 3(4):361–372. https://doi.org/10.1007/s42242-020-00085-5