Three-dimensional printing of smart constructs using stimuli-responsive biomaterials: A future direction of precision medicine
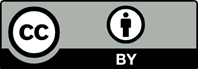
Three-dimensional (3D) printing, which is a valuable technique for the fabrication of tissue-engineered constructs and biomedical devices with complex architectures, has brought about considerable progress in regenerative medicine, drug delivery, and diagnosis of diseases. However, because of the static and inanimate properties of conventional 3D-printed structures, it is difficult to use them in therapies for active and precise medicine, such as improved tissue regeneration, targeted or controlled drug delivery, and advanced pathophysiological monitoring. The integration of stimuli-responsive biomaterials into 3D printing provides a potential strategy for designing and building smart constructs that exhibit programmed functions and controllable changes in properties in response to exogenous and autogenous stimuli. These features make 3D-printed smart constructs the next generation of tissue-engineered products. In this review, we introduce the prevalent 3D printing techniques (with an emphasis on the differences between 3D printing and bioprinting, and biomaterials and bioink), the working principle of each technique, and the advantages of using 3D printing for the fabrication of smart constructs. Stimuli-responsive biomaterials that are widely used for 3D printing of smart constructs are categorized, followed by a summary of their applications in tissue regeneration, drug delivery, and biosensors. Finally, the challenges and future perspectives of 3D-printed smart constructs are discussed.
1. Mandrycky C, Wang Z, Kim K, et al., 2016, 3D bioprinting for engineering complex tissues. Biotechnol Adv, 34: 422–434. https://doi.org/10.1016/j.biotechadv.2015.12.011
2. Di Somma M, Schaafsma W, Grillo E, et al., 2019, Natural histogel-based bio-scaffolds for sustaining angiogenesis in beige adipose tissue. Cells, 8: 1457. https://doi.org/10.3390/cells8111457
3. Han T, Kundu S, Nag A, et al., 2019, 3D printed sensors for biomedical applications: A review. Sensors, 19: 1706. https://doi.org/10.3390/s19071706
4. Sood N, Bhardwaj A, Mehta S, et al., 2016, Stimuli-responsive hydrogels in drug delivery and tissue engineering. Drug Deliv, 23: 748–770. https://doi.org/10.3109/10717544.2014.940091
5. Xia Y, He Y, Zhang F, et al., 2021, A review of shape memory polymers and composites: Mechanisms, materials, and applications. Adv Mater, 33: e2000713. https://doi.org/10.1002/adma.202000713
6. Pang X, Lv JA, Zhu C, et al., 2019, Photo deformable azobenzene-containing liquid crystal polymers and soft actuators. Adv Mater, 31: e1904224. https://doi.org/10.1002/adma.201904224
7. Yu X, Cheng H, Zhang M, et al., 2017, Graphene-based smart materials. Nat Rev Mater, 2: 17046. https://doi.org/10.1038/natrevmats.2017.46
8. Liu Z, Liu J, Cui X, et al., 2020, Recent advances on magnetic sensitive hydrogels in tissue engineering. Front Chem, 8: 124. https://doi.org/10.3389/fchem.2020.00124
9. Ghovvati M, Kharaziha M, Ardehali R, et al., 2022, Recent advances in designing electroconductive biomaterials for cardiac tissue engineering. Adv Healthc Mater, 11: 2200055. https://doi.org/10.1002/adhm.202200055
10. Gungor-Ozkerim PS, Inci I, Zhang YS, et al., 2018, Bioinks for 3D bioprinting: An overview. Biomater Sci, 6: 915–946. https://doi.org/10.1039/C7BM00765E
11. Gao B, Yang Q, Zhao X, et al., 2016, 4D Bioprinting for biomedical applications. Trends Biotechnol, 34: 746-756. https://doi.org/10.1016/j.tibtech.2016.03.004
12. Lu Y, Aimetti AA, Langer R, et al., 2016, Bioresponsive materials. Nat Rev Mater, 2: 16075. https://doi.org/10.1038/natrevmats.2016.75
13. Luo Y, Lin X, Chen B, et al., 2019, Cell-laden four-dimensional bioprinting using near-infrared-triggered shape-morphing alginate/polydopamine bioinks. Biofabrication, 11: 045019. https://doi.org/10.1088/1758-5090/ab39c5
14. Raza A, Hayat U, Rasheed T, et al., 2019, “Smart” materials based near-infrared light-responsive drug delivery systems for cancer treatment: A review. J Mater Res Technol, 8: 1497–1509. https://doi.org/10.1016/j.jmrt.2018.03.007
15. Izadifar M, Haddadi A, Chen X, et al., 2015, Rate-programming of nano-particulate delivery systems for smart bioactive scaffolds in tissue engineering. Nanotechnology, 26: 012001. https://doi.org/10.1088/0957-4484/26/1/012001
16. Zhang K, Wang S, Zhou C, et al., 2018, Advanced smart biomaterials and constructs for hard tissue engineering and regeneration. Bone Res, 6: 31. https://doi.org/.10.1038/s41413-018-0032-9
17. Williams DF, 2009, On the nature of biomaterials. Biomaterials, 30: 5897–5909. https://doi.org/10.1016/j.biomaterials.2009.07.027
18. Groll J, Burdick JA, Cho DW, et al., 2018, A definition of bioinks and their distinction from biomaterial inks. Biofabrication, 11: 013001. https://doi.org/10.1088/1758-5090/aaec52
19. Khoeini R, Nosrati H, Akbarzadeh A, et al., 2021, Natural and synthetic bioinks for 3D bioprinting. Adv NanoBiomed Res, 1(8): 2000097. https://doi.org/10.1002/anbr.202000097
20. Hull CW, 1986, Apparatus for Production of Three dimensional Objects by Stereolithography. California: Uvp
21. ASTM International, 2012, Standard Terminology for Additive Manufacturing Technologies: Designation F2792-12a. West Conshohocken PA: ASTM International.
22. Cho W, Job AV, Chen J, et al., 2018, A review of current clinical applications of three-dimensional printing in spine surgery. Asian Spine J, 12: 171–177. https://doi.org/10.4184/asj.2018.12.1.171
23. Melchels FP, Feijen J, Grijpma DW, 2010, A review on stereolithography and its applications in biomedical engineering. Biomaterials, 31: 6121–6130. https://doi.org/10.1016/j.biomaterials.2010.04.050
24. Maruo S, Ikuta I, 2002, Submicron stereolithography for the production of freely movable mechanisms by using singlephoton polymerization. Sens Actuators A, 100: 70–76.
25. Mu Q, Wang L, Dunn CK, et al., 2017, Digital light processing 3D printing of conductive complex structures. Addit Manuf, 18: 74–83. https://doi.org/10.1016/j.addma.2017.08.011
26. Kelly BE, Bhattacharya I, Heidari H, et al., 2019, Volumetric additive manufacturing via tomographic reconstruction. Science, 363: 1075–1079. https://doi.org/10.1126/science.aau7114
27. Layani M, Wang X, Magdassi S, 2018, Novel materials for 3D printing by photopolymerization. Adv Mater, 30: 1706344. https://doi.org/10.1002/adma.201706344
28. Karimi M, Ghasemi A, Zangabad PS, et al., 2016, Smart micro/nanoparticles in stimulus-responsive drug/gene delivery systems. Chem Soc Rev, 45: 1457–1501. https://doi.org/10.1039/C5CS00798D
29. Lee YW, Ceylan H, Yasa IC, et al., 2021, 3D-printed multi stimuli-responsive mobile micromachines. ACS Appl Mater Interfaces, 13: 12759–12766. https://doi.org/10.1021/acsami.0c18221
30. Amorim FL, Lohrengel A, Neubert V, et al., 2014, Selective laser sintering of Mo-CuNi composite to be used as EDM electrode. Rapid Prototyp J, 20: 59–68. https://doi.org/10.1108/rpj-04-2012-0035
31. Salmoria GV, Klauss P, Paggi RA, et al., 2009, Structure and mechanical properties of cellulose based scaffolds fabricated by selective laser sintering. Polym Test, 28: 648–652. https://doi.org/10.1016/j.polymertesting.2009.05.008
32. Williams JM, Adewunmi A, Schek RM, et al., 2005, Bone tissue engineering using polycaprolactone scaffolds fabricated via selective laser sintering. Biomaterials, 26: 4817–4827. https://doi.org/10.1016/j.biomaterials.2004.11.057
33. Wiria FE, Leong KF, Chua CK, et al., 2007, Poly-epsiloncaprolactone/hydroxyapatite for tissue engineering scaffold fabrication via selective laser sintering. Acta Biomater, 3: 1–12. https://doi.org/10.1016/j.actbio.2006.07.008
34. Mazzoli A, 2013, Selective laser sintering in biomedical engineering. Med Biol Eng Comput, 51: 245–256. https://doi.org/10.1007/s11517-012-1001-x
35. Subash A, Kandasubramanian B, 2020, 4D printing of shape memory polymers. Euro Polym J, 134: 109771. https://doi.org/10.1016/j.eurpolymj.2020.109771
36. Calvert P, 2001, Inkjet printing for materials and devices. Chem. Mater., 13: 3299–3305.
37. Park JY, Gao G, Jang J, et al., 2016, 3D printed structures for delivery of biomolecules and cells: tissue repair and regeneration. J Mater Chem B, 4: 7521–7539. https://doi.org/10.1039/c6tb01662f
38. Singh M, Haverinen HM, Dhagat P, et al., 2010, Inkjet printing-process and its applications. Adv Mater, 22: 673–685. https://doi.org/10.1002/adma.200901141
39. Bihar E, Wustoni S, Pappa AM, et al., 2018, A fully inkjet printed disposable glucose sensor on paper. Npj Flex Electron, 2: 30. https://doi.org/10.1038/s41528-018-0044-y
40. Yang J, Katagiri D, Mao S, et al., 2016, Inkjet printing based assembly of thermoresponsive core-shell polymer microcapsules for controlled drug release. J Mater Chem B, 4: 4156–4163. https://doi.org/10.1039/C6TB00424E
41. Belaid H, Nagarajan S, Teyssier C, et al., 2020, Development of new biocompatible 3D printed graphene oxide-based scaffolds. Mater Sci Eng C Mater Biol Appl, 110: 110595. https://doi.org/10.1016/j.msec.2019.110595
42. Mohamed OA, Masood SH, Bhowmik JL, 2015, Optimization of fused deposition modeling process parameters: A review of current research and future prospects. Adv Manuf, 3: 42–53. https://doi.org/10.1007/s40436-014-0097-7
43. Zeina I, Hutmacher DW, Tan KC, et al., 2002, Fused deposition modeling of novel scaffold architectures for tissue engineering applications. Biomaterials, 23: 1169–1185.
44. Ahn SH, Montero M, Odell D, et al., 2002, Anisotropic material properties of fused deposition modeling ABS. Rapid Prototyp J, 8: 248–257. https://doi.org/10.1108/13552540210441166
45. Murphy SV, Atala A, 2014, 3D bioprinting of tissues and organs. Nat Biotechnol, 32: 773–785. https://doi.org/10.1038/nbt.2958
46. O’Brart DP, 2014, Corneal collagen cross-linking: A review. J Optom, 7: 113–124. https://doi.org/10.1016/j.optom.2013.12.001
47. Kumar H, Kim K, 2020, Stereolithography 3D bioprinting. In: Crook JM, editor. 3D Bioprinting: Principles and Protocols. New York: Springer US. p. 93–108.
48. Feng M, Hu S, Qin W, et al., 2021, Bioprinting of a blue light-cross-linked biodegradable hydrogel encapsulating amniotic mesenchymal stem cells for intrauterine adhesion prevention. ACS Omega, 6: 23067–23075. https://doi.org/10.1021/acsomega.1c02117
49. Petta D, Armiento AR, Grijpma D, et al., 2018, 3D bioprinting of a hyaluronan bioink through enzymatic-and visible light-crosslinking. Biofabrication, 10: 044104. https://doi.org/10.1088/1758-5090/aadf58
50. Kim H, Kang B, Cui X, et al., 2021, Light-activated decellularized extracellular matrix-based bioinks for volumetric tissue analogs at the centimeter scale. Adv Funct Mater, 31: 2011252. https://doi.org/10.1002/adfm.202011252
51. Wang Y, Zhang S, Wang J, 2021, Photo-cross linkable hydrogel and its biological applications. Chinese Chem Lett, 32: 1603–1614. https://doi.org/10.1016/j.cclet.2020.11.073
52. Pahoff S, Meinert C, Bas O, et al., 2019, Effect of gelatin source and photoinitiator type on chondrocyte redifferentiation in gelatin methacryloyl-based tissue-engineered cartilage constructs. J Mater Chem B, 7: 1761–1772. https://doi.org/10.1039/c8tb02607f
53. Lim KS, Schon BS, Mekhileri NV, et al., 2016, New visiblelight photoinitiating system for improved print fidelity in gelatin-based bioinks. ACS Biomater Sci Eng, 2: 1752–1762. https://doi.org/10.1021/acsbiomaterials.6b00149
54. Wang Z, Kumar H, Tian Z, et al., 2018, Visible light photoinitiation of cell-adhesive gelatin methacryloyl hydrogels for stereolithography 3D bioprinting. ACS Appl Mater Interfaces, 10: 26859–26869. https://doi.org/10.1021/acsami.8b06607
55. Bernal P N, Delrot P, Loterie D, et al., 2019, volumetric bioprinting of complex living-tissue constructs within seconds. Adv Mater, 31: 1904209. https://doi.org/10.1002/adma.201904209
56. Guillotin B, Souquet A, Catros S, et al., 2010, Laser assisted bioprinting of engineered tissue with high cell density and microscale organization. Biomaterials, 31: 7250–7256. https://doi.org/10.1016/j.biomaterials.2010.05.055
57. Murphy SV, Skardal A, Atala A, 2013, Evaluation of hydrogels for bio-printing applications. J Biomed Mater Res A, 101: 272–284. https://doi.org/10.1002/jbm.a.34326
58. Khalil S, Wei SJ, 2007, Biopolymer deposition for freeform fabrication of hydrogel tissue constructs. Mater Sci Eng C, 27: 469–478.
59. Chang CC, Boland ED, Williams SK, et al., 2011, Direct write bioprinting three-dimensional biohybrid systems for future regenerative therapies. J Biomed Mater Res B Appl Biomater, 98: 160–170.
60. Khalil S, Sun W, 2007, Biopolymer deposition for freeform fabrication of hydrogel tissue constructs. Mater Sci Eng C, 27: 469–478. https://doi.org/10.1016/j.msec.2006.05.023
61. Kolesky DB, Truby RL, Gladman S, et al., 2014, 3D bioprinting of vascularized, heterogeneous cell-laden tissue constructs. Adv Mater, 26: 2966–2966.
62. Visser J, Peters B, J Burger T, et al., 2013, Biofabrication of multi-material anatomically shaped tissue constructs. Biofabrication, 5: 035007. https://doi.org/10.1088/1758-5082/5/3/035007
63. Nerger BA, Brun PT, Nelson CM, 2019, Microextrusion printing cell-laden networks of type I collagen with patterned fiber alignment and geometry. Soft Matter, 15: 5728–5738. https://doi.org/10.1039/C8SM02605J
64. Gong J, Schuurmans CC, Genderen AM, et al., 2020, Complexation-induced resolution enhancement of 3D-printed hydrogel constructs. Nat Commun, 11: 1267. https://doi.org/10.1038/s41467-020-14997-4
65. Schwab A, Hélary C, Richards RG, et al., 2020, Tissue mimetic hyaluronan bioink containing collagen fibers with controlled orientation modulating cell migration and alignment. Mater Today Bio, 7: 100058. https://doi.org/10.1016/j.mtbio.2020.100058
66. Sakiyama-Elbert S, Hubbell J, 2001, Functional biomaterials: Design of novel biomaterials. Ann Rev Mater Res, 31: 183–201.
67. He Y, Guo S, Liu Z, et al., 2015, Pattern transformation of thermo-responsive shape memory polymer periodic cellular structures. Int J Solids Struct, 71: 194–205.
68. Chen Q, Chen H, Zhu L, et al., 2015, Fundamentals of double network hydrogels. J Mater Chem B, 3: 3654–3676.
69. Haque MA, Kurokawa T, Gong JP, 2012, Super tough double network hydrogels and their application as biomaterials. Polymer, 53: 1805–1822.
70. Li G, Zhang H, Fortin D, et al., 2015, Poly (vinyl alcohol)–poly (ethylene glycol) double-network hydrogel: A general approach to shape memory and self-healing functionalities. Langmuir, 31: 11709–11716.
71. Wang D, Guo J, Zhang H, et al., 2015, Intelligent rubber with tailored properties for self-healing and shape memory. J Mater Chem A, 3: 12864–12872. https://doi.org/10.1039/c5ta01915j
72. Liu J, Huang Y, Kumar A, et al., 2014, pH-sensitive nano systems for drug delivery in cancer therapy. Biotechnol Adv, 32: 693–710.
73. Zhuo S, Zhang F, Yu J, et al., 2020, pH-sensitive biomaterials for drug delivery. Molecules, 25: 5649. https://doi.org/10.3390/molecules25235649
74. Shim JK, Lee YB, Lee YM, 1999, pH‐dependent permeation through polysulfone ultrafiltration membranes prepared by ultraviolet polymerization technique. J Appl Polym Sci, 74: 75–82.
75. Mukhopadhyay P, Sarkar K, Bhattacharya S, et al., 2014, Ph sensitive N-succinyl chitosan grafted polyacrylamide hydrogel for oral insulin delivery. Carbohydr Polym, 112: 627–637.
76. Xu Q, Huang W, Jiang L, et al., 2013, KGM and PMAA based pH-sensitive interpenetrating polymer network hydrogel for controlled drug release. Carbohydr Polym, 97: 565–570. https://doi.org/10.1016/j.carbpol.2013.05.007
77. Gupta P, Vermani K, Garg S, 2002, Hydrogels: from controlled release to pH-responsive drug delivery. Drug Discov Today, 7: 569–579.
78. Ullah F, Othman MB, Javed F, et al., 2015, Classification, processing and application of hydrogels: A review. Mater Sci Eng C, 57: 414–433.
79. Akhlaghi SP, Zaman M, Mohammed N, et al., 2015, Synthesis of amine functionalized cellulose nanocrystals: Optimization and characterization. Carbohydr Res, 409: 48–55. https://doi.org/10.1016/j.carres.2015.03.009.
80. Bao G, Jiang T, Ravanbakhsh H, et al., 2020, Triggered micropore-forming bioprinting of porous viscoelastic hydrogels. Mater Horizons, 7: 2336–2347. https://doi.org/10.1039/D0MH00813C
81. Yi S, Liu Q, Luo Z, et al., 2022, Micropore-forming gelatin methacryloyl (GelMA) bioink toolbox 2.0: Designable tunability and adaptability for 3D bioprinting applications. Small, 18: 2106357. https://doi.org/10.1002/smll.202106357
82. Gelmi A, Schutt CE, 2021, Stimuli‐responsive biomaterials: Scaffolds for stem cell control. Adv Healthc Mater, 10: 2001125.
83. Higgins MJ, Wallace GG, Molino PJ, et al., 2015, Conducting Polymers: Their Route to Nanobionics Applications via Atomic Force Microscopy. New York: Nova Science Publishers, Inc.
84. Du L, Li T, Jin F, et al., 2020, Design of high conductive and piezoelectric poly (3, 4-ethylenedioxythiophene)/chitosan nanofibers for enhancing cellular electrical stimulation. J Coll Interface Sci 559: 65–75.
85. Yi YT, Sun JY, Lu YW, et al., 2015, Programmable and on-demand drug release using electrical stimulation. Biomicrofluidics, 9: 022401.
86. Feng T, Ji W, Tang Q, et al., 2019, Low-fouling nanoporous conductive polymer-coated microelectrode for in vivo monitoring of dopamine in the rat brain. Anal Chem, 91: 10786–10791.
87. Kaewchingduang R, Paradee N, Sirivat A, et al., 2019, Effects of conductive polyazulene and plasticizer embedded in deproteinized natural rubber transdermal patch on electrically controlled naproxen release-permeation. Int J Pharm, 561: 296–304. https://doi.org/10.1016/j.ijpharm.2019.02.046
88. Meng S, Rouabhia M, Zhang Z, 2013, Electrical stimulation modulates osteoblast proliferation and bone protein production through heparin‐bioactivated conductive scaffolds. Bioelectromagnetics, 34: 189–199.
89. Kim DH, Richardson‐Burns SM, Hendricks JL, et al., 2007, Effect of immobilized nerve growth factor on conductive polymers: Electrical properties and cellular response. Adv Func Mater, 17: 79–86.
90. Wadhwa R, Lagenaur CF, Cui XT, 2006, Electrochemically controlled release of dexamethasone from conducting polymer polypyrrole coated electrode. J Control Rel, 110: 531–541.
91. Bao Z, Liu X, Liu Y, et al., 2016, Near-infrared light responsive inorganic nanomaterials for photothermal therapy. Asian J Pharm Sci, 11: 349–364.
92. Algorri J F, Ochoa M, Roldán-Varona P, et al., 2021, Light technology for efficient and effective photodynamic therapy: A critical review. Cancers (Basel), 13: 3484. https://doi.org/10.3390/cancers13143484
93. Alvarez‐Lorenzo C, Bromberg L, Concheiro A, 2009, Light sensitive intelligent drug delivery systems. Photochem Photobiol, 85: 848–860.
94. Mantha S, Pillai S, Khayambashi P, et al., 2019, Smart hydrogels in tissue engineering and regenerative medicine. Materials (Basel), 12: 3323.
95. Rapp TL, DeForest CA, 2020, Visible light‐responsive dynamic biomaterials: Going deeper and triggering more. Adv Healthc Mater, 9: 1901553. https://doi.org/10.1002/adhm.201901553
96. Lee TT, García JR, Paez JI, et al., 2015, Light-triggered in vivo activation of adhesive peptides regulates cell adhesion, inflammation and vascularization of biomaterials. Nat Mater, 14: 352–360. https://doi.org/10.1038/nmat4157
97. Aleksandrzak M, Kukulka W, Mijowska E, 2017, Graphitic carbon nitride/graphene oxide/reduced graphene oxide nanocomposites for photoluminescence and photocatalysis. Appl Surface Sci, 398: 56–62.
98. Du W, Jin Y, Lai S, et al., 2020, Multifunctional lightresponsive graphene-based polyurethane composites with shape memory, self-healing, and flame retardancy properties. Composites Part A Appl Sci Manuf, 128: 105686.
99. Qazi TH, Blatchley MR, Davidson MD, et al., 2022, Programming hydrogels to probe spatiotemporal cell biology. Cell Stem Cell. 29: 678–691.
100. Zheng Y, Chen Z, Jiang Q, et al., 2020, Near-infrared-light regulated angiogenesis in a 4D hydrogel. Nanoscale, 12: 13654–13661.
101. Gandavarapu NR, Azagarsamy MA, Anseth KS, 2014, Photo‐click living strategy for controlled, reversible exchange of biochemical ligands. Adv Mater, 26: 2521–2526.
102. Wylie RG, Ahsan S, Aizawa Y, et al., 2011, Spatially controlled simultaneous patterning of multiple growth factors in three dimensional hydrogels. Nat Mater, 10: 799–806. https://doi.org/10.1038/nmat3101
103. Hammer JA, West JL, 2018, Dynamic ligand presentation in biomaterials. Bioconjug Chem, 29: 2140–2149. https://doi.org/10.1021/acs.bioconjchem.8b00288
104. Chiriaco F, Conversano F, Soloperto G, et al., 2013, Epithelial cell biocompatibility of silica nanospheres for contrast enhanced ultrasound molecular imaging. J Nanoparticle Res, 15: 1–13.
105. Vo TN, Kasper FK, Mikos AG, 2012, Strategies for controlled delivery of growth factors and cells for bone regeneration. Adv Drug Deliv Rev, 64: 1292–1309. https://doi.org/10.1016/j.addr.2012.01.016
106. Huebsch N, Kearney CJ, Zhao X, et al., 2014, Ultrasound triggered disruption and self-healing of reversibly cross-linked hydrogels for drug delivery and enhanced chemotherapy. Proc Natl Acad Sci, 111: 9762–9767.
107. Koetting MC, Peters JT, Steichen SD, et al., 2015, Stimulus responsive hydrogels: Theory, modern advances, and applications. Mater Sci Eng R Rep, 93: 1–49.
108. Habibi M, Foroughi S, Karamzadeh V, et al., 2022, Direct sound printing. Nat Commun, 13: 1800. https://doi.org/10.1038/s41467-022-29395-1
109. Liu Z, Liu J, Cui X, et al., 2020, Recent advances on magnetic sensitive hydrogels in tissue engineering. Front Chem, 8: 124. https://doi.org/10.3389/fchem.2020.00124
110. Manjua AC, Alves VD, Crespo JO, et al., 2019, Magnetic responsive PVA hydrogels for remote modulation of protein sorption. ACS Appl Mater Interfaces, 11: 21239–21249. https://doi.org/10.1021/acsami.9b03146
111. Chen X, Fan M, Tan H, et al., 2019, Magnetic and self-healing chitosan-alginate hydrogel encapsulated gelatin microspheres via covalent cross-linking for drug delivery. Mater Sci Eng C Mater Biol Appl, 101: 619–629. https://doi.org/10.1016/j.msec.2019.04.012
112. Farzaneh S, Hosseinzadeh S, Samanipour R, et al., 2021, MNP. J Drug Deliv Sci Technol, 64: 102525.
113. Ko ES, Kim C, Choi Y, et al., 2020, 3D printing of self-healing ferrogel prepared from glycol chitosan, oxidized hyaluronate, and iron oxide nanoparticles. Carbohydr Polym, 245: 116496. https://doi.org/10.1016/j.carbpol.2020.116496
114. Guo Z, Dong L, Xia J, et al., 2021, 3D printing unique nanoclay‐incorporated double‐network hydrogels for construction of complex tissue engineering scaffolds. Adv Healthc Mater, 10: e2100036. https://doi.org/10.1002/adhm.202100036
115. Distler T, Polley C, Shi F, et al., 2021, Electrically conductive and 3D‐printable oxidized alginate‐gelatin polypyrrole: PSS hydrogels for tissue engineering. Adv Healthc Mater, 10: 2001876.
116. Siebert L, Luna‐Cerón E, García‐Rivera LE, et al., 2021, Light
controlled growth factors release on tetrapodal ZnOincorporated 3D‐printed hydrogels for developing smart wound scaffold. Adv Funct Mater, 31: 2007555. https://doi.org/10.1002/adfm.202007555
117. Maan Z, Masri NZ, Willerth SM, 2022, Smart bioinks for the printing of human tissue models. Biomolecules, 12: 141. https://doi.org/10.3390/biom12010141
118. Tiwari G, Tiwari R, Sriwastawa B, et al., 2012, Drug delivery systems: An updated review. Int J Pharm Investig, 2: 2–11. https://doi.org/10.4103/2230-973X.96920
119. Wang M, Li W, Tang G, et al., 2021, Engineering (bio) materials through shrinkage and expansion. Adv Healthc Mater, 10: 2100380.
120. Pawar AA, Saada G, Cooperstein I, et al., 2016, High performance 3D printing of hydrogels by water-dispersible photoinitiator nanoparticles. Sci Adv, 2: e1501381. https://doi.org/10.1126/sciadv.1501381
121. Melocchi A, Uboldi M, Maroni A, et al., 2020, 3D printing by fused deposition modeling of single-and multicompartment hollow systems for oral delivery-a review. Int J Pharm, 579: 119155. https://doi.org/10.1016/j.ijpharm.2020.119155
122. Ceylan H, Yasa IC, Yasa O, et al., 2019, 3D-printed biodegradable microswimmer for theranostic cargo delivery and release. ACS Nano, 13: 3353-3362. https://doi.org/10.1021/acsnano.8b09233
123. Trampe E, Koren K, Akkineni AR, et al., 2018, Functionalized bioink with optical sensor nanoparticles for O2 imaging in 3D‐bioprinted constructs. Adv Funct Mater, 28: 1804411.
124. Iversen M, Monisha M, Agarwala S, 2022, Flexible, wearable and fully-printed smart patch for pH and hydration sensing in wounds. Int J Bioprint, 8: 447. https://doi.org/10.18063/ijb.v8i1.447
125. Dargaville TR, Farrugia BL, Broadbent JA, et al., 2013, Sensors and imaging for wound healing: A review. Biosens Bioelectron, 41: 30–42. https://doi.org/10.1016/j.bios.2012.09.029
126. Bahl S, Nagar H, Singh I, et al., 2020, Smart materials types, properties and applications: A review. Mater Today Proc, 28: 1302–1306. https://doi.org/10.1016/j.matpr.2020.04.505
127. Yu Y, Zhang J, Liu JJ, 2013, Biomedical implementation of liquid metal ink as drawable ECG electrode and skin circuit. PLoS One, 8: e58771. https://doi.org/10.1371/journal.pone.0058771
128. Gantenbein S, Masania K, Woigk W, et al., 2018, Threedimensional printing of hierarchical liquid-crystal-polymer structures. Nature, 561: 226–230. https://doi.org/10.1038/s41586-018-0474-7
129. Mantha S, Pillai S, Khayambashi P, et al., 2019, Smart hydrogels in tissue engineering and regenerative medicine. Materials (Basel), 12: 3323. https://doi.org/10.3390/ma12203323
130. Zarek M, Layani M, Cooperstein I, et al., 2016, 3D printing of shape memory polymers for flexible electronic devices. Adv Mater, 28: 4449–4454. https://doi.org/10.1002/adma.201503132
131. Khoo ZX, Teoh JE, Liu Y, et al., 2015, 3D printing of smart materials: A review on recent progresses in 4D printing. Virtual Phys Prototyp, 10: 103–122. https://doi.org/10.1080/17452759.2015.1097054
132. Janbaz S, Hedayati R, Zadpoor AA, 2016, Programming the shape-shifting of flat soft matter: from self-rolling/selftwisting materials to self-folding origami. Mater Horizons, 3: 536–547. https://doi.org/10.1039/c6mh00195e
133. Zhao W, Huang Z, Liu L, et al., 2021, Porous bone tissue scaffold concept based on shape memory PLA/Fe3O4. Composites Sci Technol, 203: 108563. https://doi.org/10.1016/j.compscitech.2020.108563
134. Wang Y, Cui H, Wang Y, et al., 2021, 4D printed cardiac construct with aligned myofibers and adjustable curvature for myocardial regeneration. ACS Appl Mater Interfaces, 13: 12746–12758. https://doi.org/10.1021/acsami.0c17610
135. Ge Q, Sakhaei AH, Lee H, et al., 2016, Multimaterial 4D printing with tailorable shape memory polymers. Sci Rep, 6: 31110. https://doi.org/10.1038/srep31110
136. Zhao Z, Wu J, Mu X, et al., 2017, Origami by frontal photopolymerization. Sci Adv, 3: e1602326. https://doi.org/10.1126/sciadv.1602326
137. Li C, Lau GC, Yuan H, et al., 2020, Fast and programmable locomotion of hydrogel-metal hybrids under light and magnetic fields. Sci Robot, 5: eabb9822. https://doi.org/10.1126/scirobotics.abb9822
138. Yang G, Wang J, Yan Y, et al., 2020, Multi-stimuli-triggered shape transformation of polymeric filaments derived from dynamic covalent block copolymers. Biomacromolecules, 21: 4159–4168. https://doi.org/10.1021/acs.biomac.0c00956
139. Won JY, Kim J, Gao G, et al., 2020, 3D printing of drug loaded multi-shell rods for local delivery of bevacizumab and dexamethasone: A synergetic therapy for retinal vascular diseases. Acta Biomater, 116: 174–185. https://doi.org/10.1016/j.actbio.2020.09.015
140. Ruskowitz ER, Comerford MP, Badeau BA, et al., 2019, Logical stimuli-triggered delivery of small molecules from hydrogel biomaterials. Biomater Sci, 7: 542–546. https://doi.org/10.1039/c8bm01304g
141. Yang GH, Yeo M, Koo YW, et al., 2019, 4D bioprinting: Technological advances in biofabrication. Macromol Biosci, 19: e1800441. https://doi.org/10.1002/mabi.201800441
142. Huey DJ, Hu JC, Athanasiou KA, 2012, Unlike bone, cartilage regeneration remains elusive. Science, 338: 917–921. https://doi.org/10.1126/science.1222454
143. Narupai B, Smith PT, Nelson A, 2021, 4D printing of multistimuli responsive protein-based hydrogels for autonomous shape transformations. Adv Funct Mater, 31: 2011012. https://doi.org/10.1002/adfm.202011012
144. Iversen M, Monisha M, Agarwala S, 2022, Flexible, wearable and fully-printed smart patch for pH and hydration sensing in wounds. Int J Bioprint, 8: 447. https://doi.org/10.18063/ijb.v8i1.447
145. Lee A, Hudson AR, Shiwarski DJ, et al., 2019, 3D bioprinting of collagen to rebuild components of the human heart. Science, 365: 482–487. https://doi.org/10.1126/science.aav9051
146. Hassani FA, Peh WY, Gammad GG, et al., 2017, A 3D printed implantable device for voiding the bladder using shape memory alloy (SMA) actuators. Adv Sci (Weinh), 4: 1700143. https://doi.org/10.1002/advs.201700143
147. Dong SL, Han L, Du CX, et al., 2017, 3D printing of aniline tetramer-grafted-polyethylenimine and pluronic F127 composites for electroactive scaffolds. Macromol Rapid Commun, 38: 1600551. https://doi.org/10.1002/marc.201600551
148. Kaur MD, Mir B, Noor A, et al., 2021, 4D printing: the dawn of “smart” drug delivery systems and biomedical applications. J Drug Deliv Ther, 11: 131–137. https://doi.org/10.22270/jddt.v11i5-S.5068
149. Xu L, Zhang W, Park HB, et al., 2019, Indocyanine green and poly I:C containing thermo-responsive liposomes used in immune-photothermal therapy prevent cancer growth and metastasis. J Immunother Cancer, 7: 220. https://doi.org/10.1186/s40425-019-0702-1
150. Zhu L, Zhou Z, Mao H, et al., 2017, Magnetic nanoparticles for precision oncology: Theranostic magnetic iron oxide nanoparticles for image-guided and targeted cancer therapy. Nanomedicine (Lond), 12: 73–87. https://doi.org/10.2217/nnm-2016-0316
151. Xu Y, Wu X, Guo X, et al., 2017, The boom in 3D-printed sensor technology. Sensors (Basel), 17: 1166. https://doi.org/10.3390/s17051166
152. Kirillova A, Maxson R, Stoychev G, et al., 2017, 4D biofabrication using shape-morphing hydrogels. Adv Mater, 29: 1703443. https://doi.org/10.1002/adma.201703443
153. Wang Y, Miao Y, Zhang J, et al., 2018, Three-dimensional printing of shape memory hydrogels with internal structure for drug delivery. Mater Sci Eng C Mater Biol Appl, 84: 44–51. https://doi.org/10.1016/j.msec.2017.11.025
154. Gudapati H, Dey M, Ozbolat I, 2016, A comprehensive review on droplet-based bioprinting: Past, present and future. Biomaterials, 102: 20–42. https://doi.org/10.1016/j.biomaterials.2016.06.012
155. Christensen K, Xu C, Chai W, et al., 2015, Freeform inkjet printing of cellular structures with bifurcations. Biotechnol Bioeng, 112: 1047–1055. https://doi.org/10.1002/bit.25501
156. Cui X, Breitenkamp K, Finn MG, et al., 2012, Direct human cartilage repair using three-dimensional bioprinting technology. Tissue Eng Part A, 18: 1304–1312. https://doi.org/10.1089/ten.TEA.2011.0543
157. Heinrich MA, Liu W, Jimenez A, et al., 2019, 3D Bioprinting: From benches to translational applications. Small, 15: e1805510. https://doi.org/10.1002/smll.201805510
158. Mandrycky C, Wang Z, Kim K, et al., 2016, 3D bioprinting for engineering complex tissues. Biotechnol Adv, 34: 422–434://doi.org/10.1016/j.biotechadv.2015.12.011
159. Pedde RD, Mirani B, Navaei A, et al., 2017, Emerging biofabrication strategies for engineering complex tissue constructs. Adv Mater, 29: 1606061. https://doi.org/10.1002/adma.201606061
160. Malda J, Visser J, Melchels FP, et al., 2013, 25th anniversary article: Engineering hydrogels for biofabrication. Adv Mater, 25: 5011–5028. https://doi.org/10.1002/adma.201302042
161. Duan B, Hockaday LA, Kang KH, et al., 2012, 3D Bioprinting of heterogeneous aortic valve conduits with alginate/gelatin hydrogels. J Biomed Mater Res A, 101 : 1255–1264. https://doi.org/10.1002/jbm.a.34420
162. Chang R, Nam J, Sun W, 2008, Effects of dispensing pressure and nozzle diameter on cell survival from solid freeform fabrication-based direct cell writing. Tissue Eng Part A, 14: 41–48. https://doi.org/10.1089/ten.a.2007.0004
163. Hopp B, Smausz T, Kresz N, et al., 2005, Survival and proliferative ability of various living cell types after laserinduced forward transfer. Tissue Eng, 11: 1817–1823://doi.org/10.1089/ten.2005.11.1817
164. Schiele NR, Corr DT, Huang Y, et al., 2010, Laser-based direct-write techniques for cell printing. Biofabrication, 2: 032001. https://doi.org/10.1088/1758-5082/2/3/032001
165. Miao S, Nowicki M, Cui H, et al., 2019, 4D anisotropic skeletal muscle tissue constructs fabricated by staircase effect strategy. Biofabrication, 11: 035030. https://doi.org/10.1088/1758-5090/ab1d07
166. Hendrikson WJ, Rouwkema J, Clementi F, et al., 2017, Towards 4D printed scaffolds for tissue engineering: Exploiting 3D shape memory polymers to deliver time controlled stimulus on cultured cells. Biofabrication, 9: 031001. https://doi.org/10.1088/1758-5090/aa8114
167. Senatov FS, Niaza KV, Zadorozhnyy MY, et al., 2016, Mechanical properties and shape memory effect of 3D-printed PLA-based porous scaffolds. J Mech Behav Biomed Mater, 57: 139–148. https://doi.org/10.1016/j.jmbbm.2015.11.036
168. Miao S, Zhu W, Castro NJ, et al., 2016, 4D printing smart biomedical scaffolds with novel soybean oil epoxidized acrylate. Sci Rep, 6: 27226. https://doi.org/10.1038/srep27226
169. Miao S, Cui H, Nowicki M, et al., 2018, Photolithographicstereolithographic- tandem fabrication of 4D smart scaffolds for improved stem cell cardiomyogenic differentiation. Biofabrication, 10: 035007. https://doi.org/10.1088/1758-5090/aabe0b
170. Banche-Niclot F, Montalbano G, Fiorilli S, et al., 2021, PEG coated large mesoporous silicas as smart platform for protein delivery and their use in a collagen-based formulation for 3D printing. Int J Mol Sci, 22: 1718. https://doi.org/10.3390/ijms22041718
171. Okwuosa TC, Pereira BC, Arafat B, et al., 2017, Fabricating a shell-core delayed release tablet using dual FDM 3D printing for patient-centred therapy. Pharm Res, 34: 427–437. https://doi.org/10.1007/s11095-016-2073-3
172. Mirani B, Pagan E, Currie B, et al., 2017, An advanced multifunctional hydrogel-based dressing for wound monitoring and drug delivery. Adv Healthc Mater, 6: 1700718. https://doi.org/10.1002/adhm.201700718
173. Sayyar S, Bjorninen M, Haimi S, et al., 2016, UV cross-linkable graphene/poly(trimethylene Carbonate) composites for 3D printing of electrically conductive scaffolds. ACS Appl Mater Interfaces, 8: 31916–31925. https://doi.org10.1021/acsami.6b09962
174. Yang H, Leow WR, Wang T, et al., 2017, 3D printed photoresponsive devices based on shape memory composites. Adv Mater, 29: 1701627. https://doi.org/10.1002/adma.201701627
175. Gupta MK, Meng F, Johnson BN, et al., 2015, 3D printed programmable release capsules. Nano Lett, 15: 5321–5329. https://doi.org/10.1021/acs.nanolett.5b01688
176. Wei H, Zhang Q, Yao Y, et al., 2017, Direct-write fabrication of 4D active shape-changing structures based on a shape memory polymer and its nanocomposite. ACS Appl Mater Interfaces, 9: 876–883. https://doi.org10.1021/acsami.6b12824
177. Zhang J, Zhao S, Zhu M, et al., 2014, 3D-printed magnetic Fe3O4/MBG/PCL composite scaffolds with multifunctionality of bone regeneration, local anticancer drug delivery and hyperthermia. J Mater Chem B, 2: 7583–7595. https://doi.org/10.1039/C4TB01063A
178. D’Amora U, Russo T, Gloria A, et al., 2017, 3D additive manufactured nanocomposite magnetic scaffolds: Effect of the application mode of a time-dependent magnetic field on hMSCs behavior. Bioactive Mater, 2: 138–145. https://doi.org/10.1016/j.bioactmat.2017.04.003
179. Betsch M, Cristian C, Lin YY, et al., 2018, Incorporating 4D into bioprinting: Real-time magnetically directed collagen fiber alignment for generating complex multilayered tissues. Advanced Healthcare Materials, 7(21): 1800894. https://doi.org/10.1002/adhm.201800894
180. Zhou X, Castro NJ, Zhu W, et al., 2016, Improved human bone marrow mesenchymal stem cell osteogenesis in 3D bioprinted tissue scaffolds with low intensity pulsed ultrasound stimulation. Sci Rep, 6: 32876. https://doi.org/10.1038/srep32876