Up-to-date progress in bioprinting of bone tissue
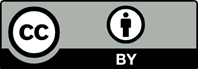
The major apparatuses used for three-dimensional (3D) bioprinting include extrusion-based, droplet-based, and laser-based bioprinting. Numerous studies have been proposed to fabricate bioactive 3D bone tissues using different bioprinting techniques. In addition to the development of bioinks and assessment of their printability for corresponding bioprinting processes, in vitro and in vivo success of the bioprinted constructs, such as their mechanical properties, cell viability, differentiation capability, immune responses, and osseointegration, have been explored. In this review, several major considerations, challenges, and potential strategies for bone bioprinting have been deliberated, including bioprinting apparatus, biomaterials, structure design of vascularized bone constructs, cell source, differentiation factors, mechanical properties and reinforcement, hypoxic environment, and dynamic culture. In addition, up-to-date progress in bone bioprinting is summarized in detail, which uncovers the immense potential of bioprinting in re-establishing the 3D dynamic microenvironment of the native bone. This review aims to assist the researchers to gain insights into the reconstruction of clinically relevant bone tissues with appropriate mechanical properties and precisely regulated biological behaviors.
1. Eliaz N, Metoki N, 2017, Calcium phosphate bioceramics: a review of their history, structure, properties, coating technologies and biomedical applications. Materials (Basel), 10: 334. https://doi.org/10.3390/ma10040334
2. Fratzl P, Weinkamer R, 2007, Nature’s hierarchical materials. Prog Mater Sci, 52: 1263–1334. https://doi.org/10.1016/j.pmatsci.2007.06.001
3. Koester KJ, Ager J, Ritchie R, 2008, The true toughness of human cortical bone measured with realistically short Cracks. Nat Mater, 7: 672–677. https://doi.org/10.1038/nmat2221
4. Rho JY, Kuhn-Spearing L, Zioupos P, 1998, Mechanical properties and the hierarchical structure of bone. Med Eng Phys, 20: 92–102. https://doi.org/10.1016/s1350-4533(98)00007-1
5. Ke P, Jiao XN, Ge XH, et al., 2014, From macro to micro: Structural biomimetic materials by electrospinning. RSC Adv, 4: 39704–39724. https://doi.org/10.1039/C4RA05098C
6. Vallet-Regí M, Navarrete DA, Arcos D, 2008, Biomimetic nanoceramics in clinical use: from materials to applications. London, UK: Royal society of chemistry.
7. Florencio-Silva R, Sasso GR, Sasso-Cerri E, et al., 2015, Biology of bone tissue: Structure, function, and factors that influence bone cells. Biomed Res Int, 2015: 421746. https://doi.org/10.1155/2015/421746
8. Murugan R, Ramakrishna S, 2005, Development of nanocomposites for bone grafting. Compos Sci Technol, 65: 2385–2406.
9. Bonfield W, Wang M, Tanner K, 1998, Interfaces in analogue biomaterials. Acta Mater, 46: 2509–2518.
10. Atala A, 2000, Tissue engineering for bladder substitution. World Journal of Urology, 18: 364–370. https://doi.org/10.1007/s003450000152
11. Melchels F, Domingos M, Klein TJ, et al., 2012, Additive manufacturing of tissues and organ. Prog Polym Sci, 37: 1079–1104.
12. Bigham A, Foroughi F, Ghomi ER, et al., 2020, The journey of multifunctional bone scaffolds fabricated from traditional toward modern techniques. Bio Des Manuf, 3: 281–306.
13. Ashammakhi N, Hasan A, Kaarela O, et al., 2019, Advancing frontiers in bone bioprinting. Adv Healthc Mater, 8: 1801048.
14. Lee JM, Yeong WY, 2016, Design and printing strategies in 3D bioprinting of cell-hydrogels: A review. Adv Healthc Mater, 5: 2856–2865. https://doi.org/10.1002/adhm.201600435
15. Gudapati H, Dey M, Ozbolat I, 2016, A comprehensive review on droplet-based bioprinting: Past, present and future. Biomaterials, 102: 20–42. https://doi.org/10.1016/j.biomaterials.2016.06.012
16. Koch L, Gruene M, Unger C, et al., 2013, Laser assisted cell printing. Curr Pharm Biotechnol, 14: 91–97.
17. Guillotin B, Ali M, Ducom A, et al., 2013, Laser-assisted bioprinting for tissue engineering. In: Forgacs g, sun w, editors. Biofabrication micro and nano-fabrication printing patterning assem. Boston: william andrew publishing. p95–p118.
18. Ayan B, Heo DN, Zhang Z, et al., 2020, Aspiration-assisted bioprinting for precise positioning of biologics. Sci Adv, 6: eaaw5111. https://doi.org/10.1126/sciadv.aaw5111
19. Lee KY, Mooney DJ, 2012, Alginate: properties and biomedical applications. Prog Polym Sci, 37: 106–126. https://doi.org/10.1016/j.progpolymsci.2011.06.003
20. Funakoshi T, Majima T, Iwasaki N, et al., 2005, Application of tissue engineering techniques for rotator cuff regeneration using a chitosan-based hyaluronan hybrid fiber scaffold. Am J Sports Med, 33: 1193–1201. https://doi.org/10.1177/0363546504272689
21. Laranjeira M, Domingues RM, Costa-Almeida R, et al., 2017, 3D mimicry of native-tissue-fiber architecture guides tendon-derived cells and adipose stem cells into artificial tendon constructs. Small, 13: 1700689. https://doi.org/10.1002/smll.201700689
22. Nichol JW, Koshy ST, Bae H, et al., 2010, Cell-laden microengineered gelatin methacrylate hydrogels. Biomaterials, 31: 5536–5544. https://doi.org/10.1016/j.biomaterials.2010.03.064
23. Yue K, Trujillo-de Santiago G, Alvarez MM, et al., 2015, Synthesis, properties, and biomedical applications of gelatin methacryloyl (GelMA) hydrogels. Biomaterials, 73: 254–271. https://doi.org/10.1016/j.biomaterials.2015.08.045
24. Klotz BJ, Gawlitta D, Rosenberg AJ, et al., 2016, Gelatin-methacryloyl hydrogels: Towards biofabrication-based tissue repair. Trends Biotechnol, 34: 394–407. https://doi.org/10.1016/j.tibtech.2016.01.002
25. D O’Connell C, Di Bella C, Thompson F, et al., 2016, Development of the biopen: A handheld device for surgical printing of adipose stem cells at a chondral wound site. Biofabrication, 8: 015019. https://doi.org/10.1088/1758-5090/8/1/015019
26. Evinger AJ, Jeyakumar JM, Hook LA, et al., 2013, Osteogenic differentiation of mesenchymal stem/stromal cells within 3D bioprinted neotissues. FASEB J, 27: 193.
27. Kuss MA, Harms R, Wu S, et al., 2017, Short-term hypoxic preconditioning promotes prevascularization in 3D bioprinted bone constructs with stromal vascular fraction derived cells. RSC Adv, 7: 29312–29320. https://doi.org/10.1039/C7RA04372D
28. Schuurman W, Khristov V, Pot MW, et al., 2011, Bioprinting of hybrid tissue constructs with tailorable mechanical Properties. Biofabrication, 3: 021001. https://doi.org/10.1088/1758-5082/3/2/021001
29. Cohen DL, Malone E, Lipson H, et al., 2006, Direct freeform fabrication of seeded hydrogels in arbitrary geometries. Tissue Eng, 12: 1325–1335. https://doi.org/10.1089/ten.2006.12.1325
30. Schagemann J, Chung H, Mrosek E, et al., 2010, Poly-∈-caprolactone/gel hybrid scaffolds for cartilage tissue engineering. J Biomed Mater Res A, 93: 454–463. https://doi.org/10.1002/jbm.a.32521
31. Endres M, Hutmacher D, Salgado A, et al., 2003, Osteogenic induction of human bone marrow-derived mesenchymal progenitor cells in novel synthetic polymer-hydrogel matrices. Tissue Eng, 9: 689–702. https://doi.org/10.1089/107632703768247386
32. Park JW, Shin YC, Kang HG, et al., 2021, In Vivo analysis of post-joint-preserving surgery fracture of 3D-printed ti-6al-4v implant to treat bone Cancer. Bio Des Manuf, 4: 879–888.
33. Li X, Yuan Y, Liu L, et al., 2020, 3D printing of hydroxyapatite/ tricalcium phosphate scaffold with hierarchical porous structure for bone regeneration. Bio Des Manuf, 3: 15–29.
34. Dong Y, Duan H, Zhao N, et al., 2018, Three-dimensional printing of β-tricalcium phosphate/calcium silicate composite scaffolds for bone tissue engineering. Bio Des Manuf, 1: 146–156.
35. Zhang B, Sun H, Wu L, et al., 2019, 3D printing of calcium phosphate bioceramic with tailored biodegradation rate for skull bone tissue reconstruction. Bio Des Manuf, 2: 161–171.
36. Oladapo BI, Zahedi SA, Ismail SO, et al., 2021, 3D printing of peek-chap scaffold for medical bone implant. Bio Des Manuf, 4: 44–59.
37. Wang X, Schröder HC, Wang K, et al., 2012, Genetic, biological and structural hierarchies during sponge spicule formation: From soft sol-gels to solid 3d silica composite structures. Soft Matter, 8: 9501–9518.
38. Wiens M, Wang X, Schröder HC, et al., 2010, The role of biosilica in the osteoprotegerin/rankl ratio in human osteoblast-like cells. Biomaterials, 31: 7716–7725. https://doi.org/10.1016/j.biomaterials.2010.07.002
39. Nguyen LH, Annabi N, Nikkhah M, et al., 2012, Vascularized bone tissue engineering: Approaches for potential improvement. Tissue Eng Part B Rev, 18: 363–382. https://doi.org/10.1089/ten.TEB.2012.0012
40. Oryan A, Alidadi S, Moshiri A, et al., 2014, Bone regenerative medicine: Classic options, novel strategies, and future directions. J Orthop Surg Res, 9: 18. https://doi.org/10.1186/1749-799X-9-18
41. Wang J, Yang M, Zhu Y, et al., 2014, Phage nanofibers induce vascularized osteogenesis in 3D printed bone scaffolds. Adv Mater, 26: 4961–4966. https://doi.org/10.1002/adma.201400154
42. Wang MO, Vorwald CE, Dreher ML, et al., 2015, Evaluating 3D-printed biomaterials as scaffolds for vascularized bone tissue engineering. Adv Mater, 27: 138–144. https://doi.org/10.1002/adma.201403943
43. Whang K, Healy K, Elenz D, et al., 1999, Engineering bone regeneration with bioabsorbable scaffolds with novel microarchitecture. Tissue Eng, 5: 35–51. https://doi.org/10.1089/ten.1999.5.35
44. Lee H, Ahn S, Bonassar LJ, et al., 2013, Cell (MC3T3-E1)- printed poly (∈-caprolactone)/alginate hybrid scaffolds for tissue regeneration. Macromol Rapid Commun, 34: 142–149. https://doi.org/10.1002/marc.201200524
45. Amini AR, Laurencin CT, Nukavarapu SP, 2012, Differential analysis of peripheral blood-and bone marrow-derived endothelial progenitor cells for enhanced vascularization in bone tissue engineering. J Orthop Res, 30: 1507–1515. https://doi.org/10.1002/jor.22097
46. Samorezov JE, Alsberg E, 2015, Spatial regulation of controlled bioactive factor delivery for bone tissue engineering. Adv Drug Deliv Rev, 84: 45–67. https://doi.org/10.1016/j.addr.2014.11.018
47. Santos MI, Reis RL, 2010, Vascularization in bone tissue engineering: Physiology, current strategies, major hurdles and future challenges. Macromol Biosci, 10: 12–27. https://doi.org/10.1002/mabi.200900107
48. Kirkpatrick CJ, Fuchs S, Unger RE, 2011, Co-culture systems for vascularization-learning from nature. Adv Drug Deliv Rev, 63: 291–299. https://doi.org/10.1016/j.addr.2011.01.009
49. Brennan MA, Davaine JM, Layrolle P, 2013, Pre-vascularization of bone tissue-engineered constructs. Stem Cell Res Ther, 4: 1–3. https://doi.org/10.1186/scrt307
50. Mishra R, Roux BM, Posukonis M, et al., 2016, Effect of prevascularization on in vivo vascularization of poly (propylene fumarate)/fibrin scaffolds. Biomaterials, 77: 255–266. https://doi.org/10.1016/j.biomaterials.2015.10.026
51. Wüst S, Godla ME, Müller R, et al., 2014, Tunable hydrogel composite with two-step processing in combination with innovative hardware upgrade for cell-based three-dimensional bioprinting. Acta Biomater, 10: 630–640. https://doi.org/10.1016/j.actbio.2013.10.016
52. Byambaa B, Annabi N, Yue K, et al., 2017, Bioprinted osteogenic and vasculogenic patterns for engineering 3D bone tissue. Adv Healthc Mater, 6: 1700015. https://doi.org/10.1002/adhm.201700015
53. Cui H, Zhu W, Nowicki M, et al., 2016, Hierarchical fabrication of engineered vascularized bone biphasic constructs via dual 3D bioprinting: Integrating regional bioactive factors into architectural design. Adv Healthc Mater, 5: 2174–2181. https://doi.org/10.1002/adhm.201600505
54. Rodan SB, Imai Y, Thiede MA, et al., 1987, Characterization of a human osteosarcoma cell line (saos-2) with osteoblastic properties. Cancer Res, 47: 4961–4966.
55. Hausser HJ, Brenner RE, 2005, Phenotypic instability of saos-2 cells in long-term culture. Biochem Biophys Res Commun, 333: 216–222. https://doi.org/10.1016/j.bbrc.2005.05.097
56. Das S, Pati F, Choi YJ, et al., 2015, Bioprintable, cell-laden silk fibroin-gelatin hydrogel supporting multilineage differentiation of stem cells for fabrication of three-dimensional tissue constructs. Acta Biomater, 11: 233–246. https://doi.org/10.1016/j.actbio.2014.09.023
57. Sabapathy V, Kumar S, 2016, hiPSC-derived iMSCs: NextGen MSCs as an advanced therapeutically active cell resource for regenerative medicine. J Cell Mol Med, 20: 1571–1588. https://doi.org/10.1111/jcmm.12839
58. Fedorovich NE, Wijnberg HM, Dhert WJ, et al., 2011, Distinct tissue formation by heterogeneous printing of osteo-and endothelial progenitor cells. Tissue Eng Part A, 17: 2113–2121. https://doi.org/10.1089/ten.TEA.2011.0019
59. Gruene M, Pflaum M, Hess C, et al., 2011, Laser printing of three-dimensional multicellular arrays for studies of cell-cell and cell-environment interactions. Tissue Eng Part C Methods, 17: 973–982. https://doi.org/10.1089/ten.TEC.2011.0185
60. Merfeld-Clauss S, Gollahalli N, March KL, et al., 2010, Adipose tissue progenitor cells directly interact with endothelial cells to induce vascular network formation. Tissue Eng Part A, 16: 2953–2966. https://doi.org/10.1089/ten.tea.2009.0635
61. Wu Y, Chen L, Scott PG, et al., 2007, Mesenchymal stem cells enhance wound healing through differentiation and angiogenesis. Stem cells, 25: 2648–2659. https://doi.org/10.1634/stemcells.2007-0226
62. Pederson L, Ruan M, Westendorf JJ, et al., 2008, Regulation of bone formation by osteoclasts involves wnt/bmp signaling and the chemokine sphingosine-1-phosphate. Proc Natl Acad Sci U S A, 105: 20764–20769. https://doi.org/10.1073/pnas.0805133106
63. Guihard P, Danger Y, Brounais B, et al., 2012, Induction of osteogenesis in mesenchymal stem cells by activated monocytes/macrophages depends on oncostatin m signaling. Stem cells, 30: 762–772. https://doi.org/10.1002/stem.1040
64. Albrektsson T, Johansson C, 2001, Osteoinduction, osteoconduction and osseointegration. Eur Spine J, 10: S96–S101. https://doi.org/10.1007/s005860100282
65. Brydone A, Meek D, Maclaine S, 2010, Bone grafting, orthopaedic biomaterials, and the clinical need for bone engineering. Proc Inst Mech Eng H, 224: 1329–1343. https://doi.org/10.1243/09544119JEIM770
66. Dimitriou R, Jones E, McGonagle D, et al., 2011, Bone regeneration: Current concepts and future directions. BMC Med, 9: 1–10.
67. Fratzl P, Gupta H, Paschalis E, et al., 2004, Structure and mechanical quality of the collagen-mineral nano-composite in bone. J Mater Chem, 14: 2115–2123. https://doi.org/10.1039/B402005G
68. Kreimendahl F, Köpf M, Thiebes AL, et al., 2017, Three-dimensional printing and angiogenesis: tailored agarose-type i collagen blends comprise three-dimensional printability and angiogenesis potential for tissue-engineered substitutes. Tissue Eng Part C Methods, 23: 604–615. https://doi.org/10.1089/ten.TEC.2017.0234
69. Karageorgiou V, Meinel L, Hofmann S, et al., 2004, Bone morphogenetic protein-2 decorated silk fibroin films induce osteogenic differentiation of human bone marrow stromal cells. J Biomed Mater Res A, 71: 528–537. https://doi.org/10.1002/jbm.a.30186
70. Zhao L, Jiang S, Hantash BM, 2010, Transforming growth factor β1 induces osteogenic differentiation of murine bone marrow stromal cells. Tissue Eng Part A, 16: 725–733. https://doi.org/10.1089/ten.TEA.2009.0495
71. Fedorovich NE, De Wijn JR, Verbout AJ, et al., 2008, Three-dimensional fiber deposition of cell-laden, viable, patterned constructs for bone tissue printing. Tissue Eng Part A, 14: 127–133. https://doi.org/10.1089/ten.a.2007.0158
72. Poldervaart MT, Goversen B, De Ruijter M, et al., 2017, 3D bioprinting of methacrylated hyaluronic acid (MeHA) hydrogel with intrinsic osteogenicity. PLoS One, 12: e0177628. https://doi.org/10.1371/journal.pone.0177628
73. McBeth C, Lauer J, Ottersbach M, et al., 2017, 3D bioprinting of gelma scaffolds triggers mineral deposition by primary human osteoblasts. Biofabrication, 9: 015009. https://doi.org/10.1088/1758-5090/aa53bd
74. Demirtaş TT, Irmak G, Gümüşderelioğlu M, 2017, A bioprintable form of chitosan hydrogel for bone tissue engineering. Biofabrication, 9: 035003. https://doi.org/10.1088/1758-5090/aa7b1d
75. Neufurth M, Wang X, Schröder HC, et al., 2014, Engineering a morphogenetically active hydrogel for bioprinting of bioartificial tissue derived from human osteoblast-like saos-2 cells. Biomaterials, 35: 8810–8819. https://doi.org/10.1016/j.biomaterials.2014.07.002
76. Pan Q, Gao C, Wang Y, et al., 2020, Investigation of bone reconstruction using an attenuated immunogenicity xenogenic composite scaffold fabricated by 3D printing. Bio Des Manuf, 3: 396–409.
77. Golafshan N, Vorndran E, Zaharievski S, et al., 2020, Tough magnesium phosphate-based 3D-printed implants induce bone regeneration in an equine defect model. Biomaterials, 261: 120302. https://doi.org/10.1016/j.biomaterials.2020.120302
78. Liu D, Nie W, Li D, et al., 2019, 3D Printed PCL/SrHA scaffold for enhanced bone regeneration. Chem Eng J, 362: 269–279.
79. Sun H, Hu C, Zhou C, et al., 2020, 3D Printing of calcium phosphate scaffolds with controlled release of antibacterial functions for jaw bone repair. Mater Des, 189: 108540. https://doi.org/10.1016/j.matdes.2020.108540
80. e Silva EP, Huang B, Helaehil JV, et al., 2021, In Vivo study of conductive 3D printed pcl/mwcnts scaffolds with electrical stimulation for bone tissue engineering. Bio Des Manuf, 4: 190–202.
81. Fedorovich NE, Schuurman W, Wijnberg HM, et al., 2012, Biofabrication of osteochondral tissue equivalents by printing topologically defined, cell-laden hydrogel scaffolds. Tissue Eng Part C Methods, 18: 33–44. https://doi.org/10.1089/ten.TEC.2011.0060
82. Shoichet MS, Li RH, White ML, et al., 1996, Stability of hydrogels used in cell encapsulation: an in vitro comparison of alginate and agarose. Biotechnol Bioeng, 50: 374–381. https://doi.org/10.1002/(SICI)1097-0290(19960520)50:4<3 74::AID-BIT4>3.0.CO;2-I
83. Bendtsen ST, Quinnell SP, Wei M, 2017, Development of a novel alginate-polyvinyl alcohol-hydroxyapatite hydrogel for 3D bioprinting bone tissue engineered scaffolds. J Biomed Mater Res A, 105: 1457–1468. https://doi.org/10.1002/jbm.a.36036
84. Kim WJ, Yun HS, Kim GH, 2017, An innovative cell-laden α-tcp/collagen scaffold fabricated using a two-step printing process for potential application in regenerating hard tissues. Sci Rep, 7: 3181. https://doi.org/10.1038/s41598-017-03455-9
85. Mishra R, Basu B, Kumar A, 2009, Physical and cytocompatibility properties of bioactive glass-polyvinyl alcohol-sodium alginate biocomposite foams prepared via sol-gel processing for trabecular bone regeneration. J Mater Sci Mater Med, 20: 2493–500. https://doi.org/10.1007/s10856-009-3814-1
86. Costa JB, Silva-Correia J, Pina S, et al., 2019, Indirect printing of hierarchical patient-specific scaffolds for meniscus tissue engineering. Bio Des Manuf, 2: 225–241.
87. Wang Q, Ma Z, Wang Y, et al., 2021, Fabrication and characterization of 3D printed biocomposite scaffolds based on pcl and zirconia nanoparticles. Bio Des Manuf, 4: 60–71.
88. Askari E, Rasouli M, Darghiasi SF, et al., 2021, Reduced graphene oxide-grafted bovine serum albumin/bredigite nanocomposites with high mechanical properties and excellent osteogenic bioactivity for bone tissue engineering. Bio Des Manuf, 4: 243–257.
89. Lee CH, Rodeo SA, Fortier LA, et al., 2014, Protein-releasing polymeric scaffolds induce fibrochondrocytic differentiation of endogenous cells for knee meniscus regeneration in sheep. Sci Transl Med, 6: 266ra171. https://doi.org/10.1126/scitranslmed.3009696
90. Ding C, Qiao Z, Jiang W, et al., 2013, Regeneration of a goat femoral head using a tissue-specific, biphasic scaffold fabricated with cad/cam technology. Biomaterials, 34: 6706–6716. https://doi.org/10.1016/j.biomaterials.2013.05.038
91. Kang HW, Lee SJ, Ko IK, et al., 2016, A 3D bioprinting system to produce human-scale tissue constructs with structural integrity. Nat Biotechnol, 34: 312–319. https://doi.org/10.1038/nbt.3413
92. Raja N, Yun HS, 2016, A simultaneous 3D printing process for the fabrication of bioceramic and cell-laden hydrogel core/shell scaffolds with potential application in bone tissue regeneration. J Mater Chem B, 4: 4707–4716. https://doi.org/10.1039/C6TB00849F
93. Araldi E, Schipani E, 2010, Hypoxia, HIFs and bone development. Bone, 47: 190–196. https://doi.org/10.1016/j.bone.2010.04.606
94. Fan W, Crawford R, Xiao Y, 2010, Enhancing In Vivo vascularized bone formation by cobalt chloride-treated bone marrow stromal cells in a tissue engineered periosteum model. Biomaterials, 31: 3580–3589. https://doi.org/10.1016/j.biomaterials.2010.01.083
95. Mamalis AA, Cochran DL, 2011, The therapeutic potential of oxygen tension manipulation via hypoxia inducible factors and mimicking agents in guided bone regeneration. A Review. Arch Oral Biol, 56: 1466–1475. https://doi.org/10.1016/j.archoralbio.2011.05.001
96. Boyette LB, Creasey OA, Guzik L, et al., 2014, Human bone marrow-derived mesenchymal stem cells display enhanced clonogenicity but impaired differentiation with hypoxic preconditioning. Stem Cells Transl Med, 3: 241–254. https://doi.org/10.5966/sctm.2013-0079
97. Merceron C, Vinatier C, Portron S, et al., 2010, Differential effects of hypoxia on osteochondrogenic potential of human adipose-derived stem cells. Am J Physiol Cell Physiol, 298: C355–C364. https://doi.org/10.1152/ajpcell.00398.2009
98. Utting J, Robins S, Brandao-Burch A, et al., 2006, Hypoxia inhibits the growth, differentiation and bone-forming capacity of rat osteoblasts. Exp Cell Res, 312: 1693–1702. https://doi.org/10.1016/j.yexcr.2006.02.007
99. Haque N, Rahman MT, Abu Kasim NH, et al., 2013, Hypoxic culture conditions as a solution for mesenchymal stem cell based regenerative Therapy. ScientificWorldJournal, 2013: 632972. https://doi.org/10.1155/2013/632972
100. Baldwin J, Antille M, Bonda U, et al., 2014, In Vitro pre-vascularisation of tissue-engineered constructs a co-culture perspective. Vasc Cell, 6: 13. https://doi.org/10.1186/2045-824X-6-13
101. Tufro-McReddie A, Norwood V, Aylor K, et al., 1997, Oxygen regulates vascular endothelial growth factor-mediated vasculogenesis and tubulogenesis. Dev Biol, 183: 139–149. https://doi.org/10.1006/dbio.1997.8513
102. Shweiki D, Itin A, Soffer D, et al., 1992, Vascular endothelial growth factor induced by hypoxia may mediate hypoxia-initiated angiogenesis. Nature, 359: 843–845. https://doi.org/10.1038/359843a0
103. Sikavitsas VI, Temenoff JS, Mikos AG, 2001, biomaterials and bone mechanotransduction. Biomaterials, 22: 2581–2593. https://doi.org/10.1016/s0142-9612(01)00002-3
104. Rubin J, Rubin C, Jacobs CR, 2006, Molecular pathways mediating mechanical signaling in bone. Gene, 367: 1–16. https://doi.org/10.1016/j.gene.2005.10.028
105. Weinbaum S, Cowin S, Zeng Y, 1994, A model for the excitation of osteocytes by mechanical loading-induced bone fluid shear stresses. J Biomech, 27: 339–360. https://doi.org/10.1016/0021-9290(94)90010-8
106. Yeatts AB, Fisher JP, 2011, Bone tissue engineering bioreactors: Dynamic culture and the influence of shear Stress. Bone, 48: 171–181. https://doi.org/10.1016/j.bone.2010.09.138
107. Bilodeau K, Mantovani D, 2006, Bioreactors for tissue engineering: focus on mechanical constraints. A comparative review. Tissue Eng, 12: 2367–2383. https://doi.org/10.1089/ten.2006.12.2367
108. Bancroft GN, Sikavitsas VI, van den Dolder J, et al., 2002, Fluid flow increases mineralized matrix deposition in 3D perfusion culture of marrow stromal osteoblasts in a dose-dependent manner. Proc Natl Acad Sci U S A, 99: 12600–12605. https://doi.org/10.1073/pnas.202296599
109. Bancroft GN, Sikavitsas VI, Mikos AG, 2003, Design of a flow perfusion bioreactor system for bone tissue-engineering applications. Tissue Eng, 9: 549–554. https://doi.org/10.1089/107632703322066723
110. Loozen LD, Wegman F, Öner FC, et al., 2013, Porous bioprinted constructs in bmp-2 non-viral gene therapy for bone tissue engineering. J Mater Chem B, 1: 6619–6626. https://doi.org/10.1039/C3TB21093F
111. Cunniffe GM, Gonzalez-Fernandez T, Daly A, et al., 2017, Three-dimensional bioprinting of polycaprolactone reinforced gene activated bioinks for bone tissue engineering. Tissue Eng Part A, 23: 891–900. https://doi.org/10.1089/ten.tea.2016.0498
112. Cidonio G, Alcala-Orozco CR, Lim KS, et al., 2019, Osteogenic and angiogenic tissue formation in high fidelity nanocomposite laponite-gelatin bioinks. Biofabrication, 11: 035027. https://doi.org/10.1088/1758-5090/ab19fd
113. Cidonio G, Glinka M, Kim YH, et al., 2020, Nanoclay-based 3D printed scaffolds promote vascular ingrowth ex vivo and generate bone mineral tissue In Vitro and In Vivo. Biofabrication, 12: 035010. https://doi.org/10.1088/1758-5090/ab8753
114. Sun J, Tan H, 2013, Alginate-based biomaterials for regenerative medicine applications. Materials (Basel), 6: 1285–1309. https://doi.org/10.3390/ma6041285
115. Araiza-Verduzco F, Rodríguez-Velázquez E, Cruz H, et al., 2020, Photocrosslinked alginate-methacrylate hydrogels with modulable mechanical properties: Effect of the molecular conformation and electron density of the methacrylate reactive group. Materials (Basel), 13: 534. https://doi.org/10.3390/ma13030534
116. Wu Y, Hospodiuk M, Peng W, et al., 2018, Porous tissue strands: Avascular building blocks for scalable tissue fabrication. Biofabrication, 11: 015009. https://doi.org/10.1088/1758-5090/aaec22
117. Wehrle M, Koch F, Zimmermann S, et al., 2019, Examination of hydrogels and mesenchymal stem cell sources for bioprinting of artificial osteogenic tissues. Cell Mol Bioeng, 12: 583–597.
118. Chiesa I, De Maria C, Lapomarda A, et al., 2020, Endothelial cells support osteogenesis in an in vitro vascularized bone model developed by 3D bioprinting. Biofabrication, 12: 025013. https://doi.org/10.1088/1758-5090/ab6a1d
119. Kim H, Yang GH, Choi CH, et al., 2018, Gelatin/PVA scaffolds fabricated using a 3d-printing process employed with a low-temperature plate for hard tissue regeneration: fabrication and characterizations. Int J Biol Macromol, 120: 119–127. https://doi.org/10.1016/j.ijbiomac.2018.07.159
120. Wang X, Tolba E, Schröder HC, et al., 2014, Effect of bioglass on growth and biomineralization of saos-2 cells in hydrogel after 3D cell bioprinting. PLoS One, 9: e112497. https://doi.org/10.1371/journal.pone.0112497
121. Heo DN, Alioglu MA, Wu Y, et al., 2020, 3D bioprinting of carbohydrazide-modified gelatin into microparticle-suspended oxidized alginate for the fabrication of complex-shaped tissue constructs. ACS Appl Mater Interfaces, 12: 20295–20306. https://doi.org/10.1021/acsami.0c05096
122. Costantini M, Idaszek J, Szöke K, et al., 2016, 3D bioprinting of bm-mscs-loaded ecm biomimetic hydrogels for in vitro neocartilage formation. Biofabrication, 8: 035002. https://doi.org/10.1088/1758-5090/8/3/035002
123. Liu B, Li J, Lei X, et al., 2020, 3D-bioprinted functional and biomimetic hydrogel scaffolds incorporated with nanosilicates to promote bone healing in rat calvarial defect model. Mater Sci Eng C Mater Biol Appl, 112: 110905. https://doi.org/10.1016/j.msec.2020.110905
124. Chimene D, Miller L, Cross LM, et al., 2020, Nanoengineered osteoinductive bioink for 3D bioprinting bone tissue. ACS Appl Mater Interfaces, 12: 15976–15988. https://doi.org/10.1021/acsami.9b19037
125. Sun X, Ma Z, Zhao X, et al., 2021, Three-dimensional bioprinting of multicell-laden scaffolds containing bone morphogenic protein-4 for promoting m2 macrophage polarization and accelerating bone defect repair in diabetes mellitus. Bioact Mater, 6: 757–769. https://doi.org/10.1016/j.bioactmat.2020.08.030
126. Dubey N, Ferreira JA, Malda J, et al., 2020, Extracellular matrix/amorphous magnesium phosphate bioink for 3d bioprinting of craniomaxillofacial bone tissue. ACS Appl Mater Interfaces, 12: 23752–23763. https://doi.org/10.1021/acsami.0c05311
127. Sawkins MJ, Mistry P, Brown BN, et al., 2015, Cell and protein compatible 3d bioprinting of mechanically strong constructs for bone repair. Biofabrication, 7: 035004. https://doi.org/10.1088/1758-5090/7/3/035004
128. Ahlfeld T, Doberenz F, Kilian D, et al., 2018, Bioprinting of mineralized constructs utilizing multichannel plotting of a self-setting calcium phosphate cement and a cell-laden Bioink. Biofabrication, 10: 045002. https://doi.org/10.1088/1758-5090/aad36d
129. Zhai X, Ruan C, Ma Y, et al., 2018, 3D-bioprinted osteoblast-laden nanocomposite hydrogel constructs with induced microenvironments promote cell viability, differentiation, and osteogenesis both In Vitro and In Vivo. Adv Sci (Weinh), 5: 1700550. https://doi.org/10.1002/advs.201700550
130. Campos DF, Blaeser A, Buellesbach K, et al., 2016, Bioprinting organotypic hydrogels with improved mesenchymal stem cell remodeling and mineralization properties for bone tissue engineering. Adv Healthc Mater, 5: 1336–1345. https://doi.org/10.1002/adhm.201501033
131. Anada T, Pan CC, Stahl AM, et al., 2019, Vascularized bone-mimetic hydrogel constructs by 3D bioprinting to promote osteogenesis and angiogenesis. Int J Mol Sci, 20: 1096. https://doi.org/10.3390/ijms20051096
132. Bernal PN, Delrot P, Loterie D, et al., 2019, Volumetric bioprinting of complex living-tissue constructs within seconds. Adv Mater, 31: 1904209.
133. Heo DN, Ayan B, Dey M, et al., 2020, Aspiration-assisted bioprinting of co-cultured osteogenic spheroids for bone tissue engineering. Biofabrication, 13: 015013. https://doi.org/10.1088/1758-5090/abc1bf
134. Rukavina P, Koch F, Wehrle M, et al., 2020, In Vivo evaluation of bioprinted prevascularized bone tissue. Biotechnol Bioeng, 117: 3902-11. https://doi.org/10.1002/bit.27527
135. Ashammakhi N, Ahadian S, Xu C, et al., 2019, Bioinks and bioprinting technologies to make heterogeneous and biomimetic tissue constructs. Mater Today Bio, 1: 100008. https://doi.org/10.1016/j.mtbio.2019.100008
136. Mercado-Pagán ÁE, Stahl AM, Shanjani Y, et al., 2015, Vascularization in bone tissue engineering constructs. Ann Biomed Eng, 43: 718–729. https://doi.org/10.1007/s10439-015-1253-3
137. Ramtani S, 2008, Electro-mechanics of bone remodelling. Int J Eng Sci, 46: 1173–1182. https://doi.org/10.1016/j.ijengsci.2008.06.001
138. Moura D, Pereira RF, Gonçalves IC, 2022, Recent advances on bioprinting of hydrogels containing carbon materials. Mater Today Chem, 23: 100617. https://doi.org/10.1016/j.mtchem.2021.100617