Advanced 3D-Printing Bioinks for Articular Cartilage Repair
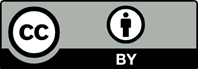
Chondral lesions caused by stressors, such as injury or inflammation, lead to osteoarthritis (OA). OA is a degenerative joint disease that has become a challenge worldwide. As the articular cartilage is incapable of self-regeneration due to the absence of vessels and nerves, novel cartilage repair techniques are urgently needed. Three-dimensional (3D) bioprinting, which allows the precise control of internal architecture and geometry of printed scaffolds, has stepped up to be a promising strategy in cartilage restoration. With regards to 3D bioprinting, bioinks with proper chemical and mechanical properties play one of the most critical roles in designing successful cartilage tissue constructs. In particular, hydrogels as 3D hydrophilic cross-linked polymer networks are highly recommended as bioinks because of their fine biocompatibility, easy fabrication, and tunable mechanical strength. Herein, we highlight the widely used polymers for hydrogel preparation and further provide a non-exhaustive overview of various functional modified additives (such as cells, drugs, bioactive factors and ceramic) to exploit the unique properties suitable for bioprinted cartilage. Finally, a prospective on future development for 3D-bioprinting in cartilage repair is elucidated in this review.
1. Messina OD, Wilman MV, Neira LF, 2019, Nutrition, Osteoarthritis and Cartilage Metabolism. Aging Clin Exp Res, 31:807–13. https://doi.org/10.1007/s40520-019-01191-w
2. Lagarto JL, Nickdel MB, Kelly DJ, et al., 2020, Autofluorescence Lifetime Reports Cartilage Damage in Osteoarthritis. Sci Rep, 10:2154. https://doi.org/10.1038/s41598-020-59219-5
3. Gourdine J, 2019, Review of Nonsurgical Treatment Guidelines for Lower Extremity Osteoarthritis. Orthop Nurs, 38:303–8. https://doi.org/10.1097/nor.0000000000000591
4. Wei W, Ma Y, Yao X, et al., 2021, Advanced Hydrogels for the Repair of Cartilage Defects and Regeneration. Bioact Mater, 6:998–1011. https://doi.org/10.1016/j.bioactmat.2020.09.030
5. Glyn-Jones S, Palmer AJ, Agricola R, et al., 2015, Osteoarthritis. Lancet, 386:376–87. https://doi.org/10.1016/s0140-6736(14)60802-3
6. Sacitharan PK, 2019, Ageing and Osteoarthritis. In: Harris JR, Korolchuk VI, editors. Biochemistry and Cell Biology of Ageing: Part II Clinical Science. Singapore: Springer. p123–59.
7. Guo T, Lembong J, Zhang LG, et al., 2017, Three-Dimensional Printing Articular Cartilage: Recapitulating the Complexity of Native Tissue. Tissue Eng Part B Rev, 23:225–36. https://doi.org/10.1089/ten.TEB.2016.0316
8. Xue JX, Gong YY, Zhou GD, et al., 2012, Chondrogenic Differentiation of Bone Marrow-derived Mesenchymal Stem Cells Induced by Acellular Cartilage Sheets. Biomaterials, 33:5832–40. https://doi.org/10.1016/j.biomaterials.2012.04.054
9. Zhang YS, Khademhosseini A, 2017, Advances in Engineering Hydrogels. Science, 356:eaaf3627. https://doi.org/10.1126/science.aaf3627
10. Mei Q, Rao J, Bei HP, et al., 2021, 3D Bioprinting Photo-Crosslinkable Hydrogels for Bone and Cartilage Repair. Int J Bioprint, 7:367. https://doi.org/10.18063/ijb.v7i3.367
11. Marques CF, Diogo GS, Pina S, et al., 2019, Collagen based Bioinks for Hard Tissue Engineering Applications: A Comprehensive Review. J Mater Sci Mater Med, 30:32. https://doi.org/10.1007/s10856-019-6234-x
12. Gillispie GJ, Han A, Uzun-Per M, et al., 2020, The Influence of Printing Parameters and Cell Density on Bioink Printing Outcomes. Tissue Eng Part A, 26:1349–58. https://doi.org/10.1089/ten.TEA.2020.0210
13. Diamantides N, Dugopolski C, Blahut E, et al., 2019, High Density Cell Seeding Affects the Rheology and Printability of Collagen Bioinks. Biofabrication, 11:045016. https://doi.org/10.1088/1758-5090/ab3524
14. Schwartz R, Malpica M, Thompson GL, et al., 2020, Cell Encapsulation in Gelatin Bioink Impairs 3D Bioprinting Resolution. J Mech Behav Biomed Mater, 103:103524. https://doi.org/10.1016/j.jmbbm.2019.103524
15. Hsu EL, Stock SR, 2020, Growth Factors, Carrier Materials, and Bone Repair. Handb Exp Pharmacol, 262:121–56. https://doi.org/10.1007/164_2020_371
16. Chen L, Liu J, Guan M, et al., 2020, Growth Factor and Its Polymer Scaffold-Based Delivery System for Cartilage Tissue Engineering. Int J Nanomedicine, 15:6097–111. https://doi.org/10.2147/ijn.S249829
17. Shirahama H, Lee BH, Tan LP, et al., 2016, Precise Tuning of Facile One-Pot Gelatin Methacryloyl (GelMA) Synthesis. Sci Rep, 6:31036. https://doi.org/10.1038/srep31036
18. Chimene D, Kaunas R, Gaharwar AK, 2020, Hydrogel Bioink Reinforcement for Additive Manufacturing: A Focused Review of Emerging Strategies. Adv Mater, 32:e1902026. https://doi.org/10.1002/adma.201902026
19. Bertlein S, Brown G, Lim KS, et al., 2017, Thiol-Ene Clickable Gelatin: A Platform Bioink for Multiple 3D Biofabrication Technologies. Adv Mater, 29:44. https://doi.org/10.1002/adma.201703404
20. Liao J, Qu Y, Chu B, et al., 2015, Biodegradable CSMA/PECA/Graphene Porous Hybrid Scaffold for Cartilage Tissue Engineering. Sci Rep, 5:9879. https://doi.org/10.1038/srep09879
21. Cui X, Li J, Hartanto Y, et al., 2020, Advances in Extrusion 3D Bioprinting: A Focus on Multicomponent Hydrogel-Based Bioinks. Adv Healthc Mater, 9:e1901648. https://doi.org/10.1002/adhm.201901648
22. Zhang YS, Haghiashtiani G, Hübscher T, et al., 2021, 3D Extrusion Bioprinting. Nat Rev Methods Prim, 1:75. https://doi.org/10.1038/s43586-021-00073-8
23. Daly AC, Freeman FE, Gonzalez-Fernandez T, et al., 2017, 3D Bioprinting for Cartilage and Osteochondral Tissue Engineering. Adv Healthc Mater, 6:22. https://doi.org/10.1002/adhm.201700298
24. Willson K, Atala A, Yoo JJ, 2021, Bioprinting Au Natural: The Biologics of Bioinks. Biomolecules, 11:1593. https://doi.org/10.3390/biom11111593
25. Zhang J, Hu Q, Wang S, et al., 2020, Digital Light Processing Based Three-dimensional Printing for Medical Applications. Int J Bioprint, 6:242. https://doi.org/10.18063/ijb.v6i1.242
26. Petta D, D’Amora U, Ambrosio L, et al., 2020, Hyaluronic Acid as a Bioink for Extrusion-based 3D Printing. Biofabrication, 12:032001. https://doi.org/10.1088/1758-5090/ab8752
27. Kim SH, Kim DY, Lim TH, et al., 2020, Silk Fibroin Bioinks for Digital Light Processing (DLP) 3D Bioprinting. In: Chun HJ, Reis RL, Motta A, Khang G. editors. Bioinspired Biomaterials: Advances in Tissue Engineering and Regenerative Medicine. Singapore: Springer. p53-66.
28. Antich C, de Vicente J, Jiménez G, et al., 2020, Bio-inspired Hydrogel Composed of Hyaluronic Acid and Alginate as a Potential Bioink for 3D Bioprinting of Articular Cartilage Engineering Constructs. Acta Biomater, 106:114–23. https://doi.org/10.1016/j.actbio.2020.01.046
29. Fraser JR, Laurent TC, Laurent UB, 1997, Hyaluronan: Its Nature, Distribution, Functions and Turnover. J Intern Med, 242:27–33. https://doi.org/10.1046/j.1365-2796.1997.00170.x
30. Evanko SP, Angello JC, Wight TN, 1999, Formation of Hyaluronan and Versican-rich Pericellular Matrix is Required for Proliferation and Migration of Vascular Smooth Muscle Cells. Arterioscler Thromb Vasc Biol, 19:1004–13. https://doi.org/10.1161/01.atv.19.4.1004
31. Abatangelo G, Vindigni V, Avruscio G, et al., 2020, Hyaluronic Acid: Redefining its Role. Cells, 9:1743. https://doi.org/10.3390/cells9071743
32. Burdick JA, Prestwich GD, 2011, Hyaluronic Acid Hydrogels for Biomedical Applications. Adv Mater, 23:H41–56. https://doi.org/10.1002/adma.201003963
33. Shu XZ, Ahmad S, Liu Y, et al., 2006, Synthesis and Evaluation of Injectable, In Situ Crosslinkable Synthetic Extracellular Matrices for Tissue Engineering. J Biomed Mater Res A, 79:902–12. https://doi.org/10.1002/jbm.a.30831
34. Vanderhooft JL, Mann BK, Prestwich GD, 2007, Synthesis and Characterization of Novel Thiol-reactive Poly(Ethylene Glycol) Cross-linkers for Extracellular-matrix-mimetic Biomaterials. Biomacromolecules, 8:2883–9. https://doi.org/10.1021/bm0703564
35. Serban MA, Prestwich GD, 2007, Synthesis of Hyaluronan Haloacetates and Biology of Novel Cross-linker-free Synthetic Extracellular Matrix Hydrogels. Biomacromolecules, 8:2821–8. https://doi.org/10.1021/bm700595s
36. Pouyani T, Prestwich GD, 1994, Functionalized Derivatives of Hyaluronic Acid Oligosaccharides: Drug Carriers and Novel Biomaterials. Bioconjug Chem, 5:339–47. https://doi.org/10.1021/bc00028a010
37. Darr A, Calabro A, 2009, Synthesis and Characterization of Tyramine-based Hyaluronan Hydrogels. J Mater Sci Mater Med, 20:33–44. https://doi.org/10.1007/s10856-008-3540-0
38. Ozbolat IT, Hospodiuk M, 2016, Current Advances and Future Perspectives in Extrusion-based Bioprinting. Biomaterials, 76:321–43. https://doi.org/10.1016/j.biomaterials.2015
39. Kesti M, Müller M, Becher J, et al., 2015, A Versatile Bioink for Three-dimensional Printing of Cellular Scaffolds Based on Thermally and Photo-triggered Tandem Gelation. Acta Biomater, 11:162–72. https://doi.org/10.1016/j.actbio.2014.09.033
40. Poldervaart MT, Goversen B, de Ruijter M, et al., 2017, 3D Bioprinting of Methacrylated Hyaluronic Acid (MeHA) Hydrogel with Intrinsic Osteogenicity. PLoS One, 12:e0177628. https://doi.org/10.1371/journal.pone.0177628
41. Lin H, Beck AM, Shimomura K, et al., 2019, Optimization of Photocrosslinked Gelatin/hyaluronic Acid Hybrid Scaffold for the Repair of Cartilage Defect. J Tissue Eng Regen Med, 13:1418–29. https://doi.org/10.1002/term.2883
42. Titan A, Schär M, Hutchinson I, et al., 2020, Growth Factor Delivery to a Cartilage-Cartilage Interface Using Platelet-Rich Concentrates on a Hyaluronic Acid Scaffold. Arthroscopy, 36:1431–40. https://doi.org/10.1016/j.arthro.2019.12.004
43. Qi X, Tong X, Pan W, et al., 2021, Recent Advances in Polysaccharide-based Adsorbents for Wastewater Treatment. J Cleaner Prod, 315:128221. https://doi.org/10.1016/j.jclepro.2021.128221
44. Axpe E, Oyen ML, 2016, Applications of Alginate-Based Bioinks in 3D Bioprinting. Int J Mol Sci, 17:1976. https://doi.org/10.3390/ijms17121976
45. Hadley DJ, Silva EA, 2019, Thaw-Induced Gelation of Alginate Hydrogels for Versatile Delivery of Therapeutics. Ann Biomed Eng, 47:1701–10. https://doi.org/10.1007/s10439-019-02282-5
46. Kabir W, Di Bella C, Choong PF, et al., 2020, Assessment of Native Human Articular Cartilage: A Biomechanical Protocol. Cartilage, 13:427S–37. https://doi.org/10.1177/1947603520973240
47. Yang X, Lu Z, Wu H, et al., 2018, Collagen-alginate as Bioink for Three-dimensional (3D) Cell Printing Based Cartilage Tissue Engineering. Mater Sci Eng C Mater Biol Appl, 83:195–201. https://doi.org/10.1016/j.msec.2017.09.002
48. Rastogi P, Kandasubramanian B, 2019, Review of Alginatebased Hydrogel Bioprinting for Application in Tissue Engineering. Biofabrication, 11:042001. https://doi.org/10.1088/1758-5090/ab331e
49. Reakasame S, Boccaccini AR, 2018, Oxidized Alginate-Based Hydrogels for Tissue Engineering Applications: A Review. Biomacromolecules, 19:3–21. https://doi.org/10.1021/acs.biomac.7b01331
50. Luo Y, Li Y, Qin X, et al., 2018, 3D Printing of Concentrated Alginate/Gelatin Scaffolds with Homogeneous Nano Apatite Coating for Bone Tissue Engineering. Mater Des, 146:12–9. https://doi.org/10.1016/j.matdes.2018.03.002
51. Fedorovich NE, Schuurman W, Wijnberg HM, et al., 2012, Biofabrication of Osteochondral Tissue Equivalents by Printing Topologically Defined, Cell-laden Hydrogel Scaffolds. Tissue Eng Part C Methods, 18:33–44. https://doi.org/10.1089/ten.TEC.2011.0060
52. Bakarich SE, Gorkin R 3rd, in het Panhuis M, et al., 2014, Three-dimensional Printing Fiber Reinforced Hydrogel Composites. ACS Appl Mater Interfaces, 6:15998–6006. https://doi.org/10.1021/am503878d
53. Kundu J, Shim JH, Jang J, et al., 2015, An Additive Manufacturing-based PCL-alginate-chondrocyte Bioprinted Scaffold for Cartilage Tissue Engineering. J Tissue Eng Regen Med, 9:1286–97. https://doi.org/10.1002/term.1682
54. Kosik-Kozioł A, Costantini M, Bolek T, et al., 2017, PLA Short Sub-micron Fiber Reinforcement of 3D Bioprinted Alginate Constructs for Cartilage Regeneration. Biofabrication, 9:044105. https://doi.org/10.1088/1758-5090/aa90d7
55. Olate-Moya F, Arens L, Wilhelm M, et al., 2020, Chondroinductive Alginate-Based Hydrogels Having Graphene Oxide for 3D Printed Scaffold Fabrication. ACS Appl Mater Interfaces, 12:4343–57. https://doi.org/10.1021/acsami.9b22062
56. Schwarz S, Kuth S, Distler T, et al., 2020, 3D Printing and Characterization of Human Nasoseptal Chondrocytes Laden Dual Crosslinked Oxidized Alginate-gelatin Hydrogels for Cartilage Repair Approaches. Mater Sci Eng C Mater Biol Appl, 116:111189. https://doi.org/10.1016/j.msec.2020.111189
57. Depalle B, Qin Z, Shefelbine SJ, et al., 2015, Influence of Cross-link Structure, Density and Mechanical Properties in the Mesoscale Deformation Mechanisms of Collagen Fibrils. J Mech Behav Biomed Mater, 52:1–13. https://doi.org/10.1016/j.jmbbm.2014.07.008
58. Bellis SL, 2011, Advantages of RGD Peptides for Directing Cell Association with Biomaterials. Biomaterials, 32:4205–42. https://doi.org/10.1016/j.biomaterials.2011.02.029
59. Adamiak K, Sionkowska A, 2020, Current Methods of Collagen Cross-linking: Review. Int J Biol Macromol, 161:550–60. https://doi.org/10.1016/j.ijbiomac.2020.06.075
60. Lee H, Yang GH, Kim M, et al., 2018, Fabrication of Micro/Nanoporous Collagen/dECM/Silk-fibroin Biocomposite Scaffolds Using a Low Temperature 3D Printing Process for Bone Tissue Regeneration. Mater Sci Eng C Mater Biol Appl, 84:140–7. https://doi.org/10.1016/j.msec.2017.11.013
61. Shim JH, Jang KM, Hahn SK, et al., 2016, Three-dimensional Bioprinting of Multilayered Constructs Containing Human Mesenchymal Stromal Cells for Osteochondral Tissue Regeneration in the Rabbit Knee Joint. Biofabrication, 8:014102. https://doi.org/10.1088/1758-5090/8/1/014102
62. Stratesteffen H, Köpf M, Kreimendahl F, et al., 2017, GelMA collagen Blends Enable Drop-on-demand 3D Printablility and Promote Angiogenesis. Biofabrication, 9:045002. https://doi.org/10.1088/1758-5090/aa857c
63. Lee J, Yeo M, Kim W, et al., 2018, Development of a Tannic Acid Cross-linking Process for Obtaining 3D Porous Cellladen Collagen Structure. Int J Biol Macromol, 110:497–503. https://doi.org/10.1016/j.ijbiomac.2017
64. Wang C, Yue H, Huang W, et al., 2020, Cryogenic 3D Printing of Heterogeneous Scaffolds with Gradient Mechanical Strengths and Spatial Delivery of Osteogenic Peptide/TGF-β1 for Osteochondral Tissue Regeneration. Biofabrication, 12:025030. https://doi.org/10.1088/1758-5090/ab7ab5
65. Tong X, Pan W, Su T, et al., 2020, Recent Advances in Natural Polymer-based Drug Delivery Systems. React Funct Polym, 148:104501. https://doi.org/10.1016/j.reactfunctpolym.2020.104501
66. Catoira MC, Fusaro L, Di Francesco D, et al., 2019, Overview of Natural Hydrogels for Regenerative Medicine Applications. J Mater Sci Mater Med, 30:115. https://doi.org/10.1007/s10856-019-6318-7
67. Kim HJ, Kim MK, Lee KH, et al., 2017, Effect of Degumming Methods on Structural Characteristics and Properties of Regenerated Silk. Int J Biol Macromol, 104:294–302. https://doi.org/10.1016/j.ijbiomac.2017.06.019
68. Rasheed T, Bilal M, Zhao Y, et al., 2019, Physiochemical Characteristics and Bone/Cartilage Tissue Engineering Potentialities of Protein-based Macromolecules a Review. Int J Biol Macromol, 121:13–22. https://doi.org/10.1016/j.ijbiomac.2018
69. Singh YP, Bandyopadhyay A, Mandal BB, 2019, 3D Bioprinting Using Cross-Linker-Free Silk-Gelatin Bioink for Cartilage Tissue Engineering. ACS Appl Mater Interfaces, 11:33684–96. https://doi.org/10.1021/acsami.9b11644
70. Vepari C, Kaplan DL, 2007, Silk as a Biomaterial. Prog Polym Sci, 32:991–1007. https://doi.org/10.1016/j.progpolymsci.2007.05.013
71. Shi W, Sun M, Hu X, et al., 2017, Structurally and Functionally Optimized Silk-Fibroin-Gelatin Scaffold Using 3D Printing to Repair Cartilage Injury In Vitro and In Vivo. Adv Mater, 29:1701089. https://doi.org/10.1002/adma.201701089
72. Kim SH, Yeon YK, Lee JM, et al., 2018, Precisely Printable and Biocompatible Silk Fibroin Bioink for Digital Light Processing 3D Printing. Nat Commun, 9:1620. https://doi.org/10.1038/s41467-018-03759-y
73. Yue K, Trujillo-de Santiago G, Alvarez MM, et al., 2015, Synthesis, Properties, and Biomedical Applications of Gelatin Methacryloyl (GelMA) Hydrogels. Biomaterials, 73:254–71. https://doi.org/10.1016/j.biomaterials.2015.08.045
74. Chen YC, Lin RZ, Qi H, et al., 2012, Functional Human Vascular Network Generated in Photocrosslinkable Gelatin Methacrylate Hydrogels. Adv Funct Mater, 22:2027–39. https://doi.org/10.1002/adfm.201101662
75. Koshy ST, Ferrante TC, Lewin SA, et al., 2014, Injectable, Porous, and Cell-responsive Gelatin Cryogels. Biomaterials, 35:2477–87. https://doi.org/10.1016/j.biomaterials.2013.11.044
76. Wei D, Xiao W, Sun J, et al., 2015, A Biocompatible Hydrogel with Improved Stiffness and Hydrophilicity for Modular Tissue Engineering Assembly. J Mater Chem B, 3:2753–63. https://doi.org/10.1039/c5tb00129c
77. Liu Y, Chan-Park MB, 2010, A Biomimetic Hydrogel Based on Methacrylated Dextran-graft-lysine and Gelatin for 3D Smooth Muscle Cell Culture. Biomaterials, 31:1158–70. https://doi.org/10.1016/j.biomaterials.2009
78. Wang Y, Ma M, Wang J, et al., 2018, Development of a Photo-Crosslinking, Biodegradable GelMA/PEGDA Hydrogel for Guided Bone Regeneration Materials. Materials (Basel), 11:1345. https://doi.org/10.3390/ma11081345
79. Mobasheri A, Rayman MP, Gualillo O, et al., 2017, The Role of Metabolism in the Pathogenesis of Osteoarthritis. Nat Rev Rheumatol, 13:302–11. https://doi.org/10.1038/nrrheum.2017.50
80. Chen P, Zheng L, Wang Y, et al., 2019, Desktop stereolithography 3D Printing of a Radially Oriented Extracellular Matrix/Mesenchymal Stem Cell Exosome Bioink for Osteochondral Defect Regeneration. Theranostics, 9:2439–59. https://doi.org/10.7150/thno.31017
81. Lam T, Dehne T, Krüger JP, et al., 2019, Photopolymerizable Gelatin and Hyaluronic Acid for Stereolithographic 3D Bioprinting of Tissue-engineered Cartilage. J Biomed Mater Res B Appl Biomater, 107:2649–57. https://doi.org/10.1002/jbm.b.34354
82. Luo C, Xie R, Zhang J, et al., 2020, Low-Temperature Three-Dimensional Printing of Tissue Cartilage Engineered with Gelatin Methacrylamide. Tissue Eng Part C Methods, 26:306–16. https://doi.org/10.1089/ten.TEC.2020.0053
83. Irmak G, Gümüşderelioğlu M, 2020, Photo-activated Platelet-rich Plasma (PRP)-based Patient-specific Bio-ink for Cartilage Tissue Engineering. Biomed Mater, 15:0650. https://doi.org/10.1088/1748-605X/ab9e46
84. Chin SY, Poh YC, Kohler AC, et al., 2018, An Additive Manufacturing Technique for the Facile and Rapid Fabrication of Hydrogel-based Micromachines with Magnetically Responsive Components. J Vis Exp, 137:56727. https://doi.org/10.3791/56727
85. Arcaute K, Mann B, Wicker R, 2010, Stereolithography of Spatially Controlled Multi-material Bioactive Poly(Ethylene Glycol) Scaffolds. Acta Biomater, 6:1047–54. https://doi.org/10.1016/j.actbio.2009.08.017
86. Bryant SJ, Anseth KS, 2002, Hydrogel Properties Influence ECM Production by Chondrocytes Photo encapsulated in Poly(Ethylene Glycol) Hydrogels. J Biomed Mater Res, 59:63–72. https://doi.org/10.1002/jbm.1217
87. Sharma B, Fermanian S, Gibson M, et al., 2013, Human Cartilage Repair with a Photoreactive Adhesive-hydrogel Composite. Sci Transl Med, 5:167ra166. https://doi.org/10.1126/scitranslmed.3004838
88. Gaharwar AK, Dammu SA, Canter JM, et al., 2011, Highly Extensible, Tough, and Elastomeric Nanocomposite Hydrogels from Poly(Ethylene Glycol) and Hydroxyapatite Nanoparticles. Biomacromolecules, 12:1641–50. https://doi.org/10.1021/bm200027z
89. Gaharwar AK, Kishore V, Rivera C, et al., 2012, Physically Crosslinked Nanocomposites from Silicate-crosslinked PEO: Mechanical Properties and Osteogenic Differentiation of Human Mesenchymal Stem Cells. Macromol Biosci, 12:779–93. https://doi.org/10.1002/mabi.201100508
90. Zhang W, Lian Q, Li D, et al., 2014, Cartilage Repair and Subchondral Bone Migration Using 3D Printing Osteochondral Composites: A One-year-period Study in Rabbit Trochlea. Biomed Res Int, 2014:746138. https://doi.org/10.1155/2014/746138
91. Treccani L, Klein TY, Meder F, et al., 2013, Functionalized Ceramics for Biomedical, Biotechnological and Environmental Applications. Acta Biomater, 9:7115–50. https://doi.org/10.1016/j.actbio.2013.03.036
92. Gao G, Schilling AF, Hubbell K, et al., 2015, Improved Properties of Bone and Cartilage Tissue from 3D Inkjet bioprinted Human Mesenchymal Stem Cells by Simultaneous Deposition and Photocrosslinking in PEG-GelMA. Biotechnol Lett, 37:2349–55. https://doi.org/10.1007/s10529-015-1921-2
93. Qiao Z, Lian M, Han Y, et al., 2021, Bioinspired Stratified Electrowritten Fiber-reinforced Hydrogel Constructs with Layer-specific Induction Capacity for Functional Osteochondral Regeneration. Biomaterials, 266:120385. https://doi.org/10.1016/j.biomaterials.2020.120385
94. Kade JC, Dalton PD, 2021, Polymers for Melt Electrowriting. Adv Healthc Mater, 10:e2001232. https://doi.org/10.1002/adhm.202001232
95. Jiang S, Guo W, Tian G, et al., 2020, Clinical Application Status of Articular Cartilage Regeneration Techniques: Tissue-Engineered Cartilage Brings New Hope. Stem Cells Int, 2020:5690252. https://doi.org/10.1155/2020/5690252
96. Shive MS, Hoemann CD, Restrepo A, et al., 2006, BSTCarGel: In Situ ChondroInduction for Cartilage Repair. Oper Techn Orthop, 16:271–8. https://doi.org/10.1053/j.oto.2006.08.001
97. Sahana TG, Rekha PD, 2018, Biopolymers: Applications in Wound Healing and Skin Tissue Engineering. Mol Biol Rep, 45:2857–67. https://doi.org/10.1007/s11033-018-4296-3
98. Daly AC, Critchley SE, Rencsok EM, et al., 2016, A Comparison of Different Bioinks for 3D Bioprinting of Fibrocartilage and Hyaline Cartilage. Biofabrication, 8:045002. https://doi.org/10.1088/1758-5090/8/4/045002
99. Dhand AP, Galarraga JH, Burdick JA, 2021, Enhancing Biopolymer Hydrogel Functionality through Interpenetrating Networks. Trends Biotechnol, 39:519–38. https://doi.org/10.1016/j.tibtech.2020.08.007
100. Shojarazavi N, Mashayekhan S, Pazooki H, et al., 2021, Alginate/Cartilage Extracellular Matrix-based Injectable Interpenetrating Polymer Network Hydrogel for Cartilage Tissue Engineering. J Biomater Appl, 36:803–17. https://doi.org/10.1177/08853282211024020
101. D’Angelo M, Billings PC, Pacifici M, et al., 2001, Authentic Matrix Vesicles Contain Active Metalloproteases (MMP). A Role for Matrix Vesicle-associated MMP-13 in Activation of Transforming Growth Factor-beta. J Biol Chem, 276:11347–53. https://doi.org/10.1074/jbc.M009725200
102. Jahangir S, Eglin D, Pötter N, et al., 2020, Inhibition of Hypertrophy and Improving Chondrocyte Differentiation by MMP-13 Inhibitor Small Molecule Encapsulated in Alginate chondroitin Sulfate-platelet Lysate Hydrogel. Stem Cell Res Ther, 11:436. https://doi.org/10.1186/s13287-020-01930-1
103. Stefani RM, Barbosa S, Tan AR, et al., 2020, Pulsed Electromagnetic Fields Promote Repair of Focal Articular Cartilage Defects with Engineered Osteochondral Constructs. Biotechnol Bioeng, 117:1584–96. https://doi.org/10.1002/bit.27287
104. Kino-Oka M, Maeda Y, Sato Y, et al., 2009, Morphological Evaluation of Chondrogenic Potency in Passaged Cell Populations. J Biosci Bioeng, 107:544–51. https://doi.org/10.1016/j.jbiosc.2008.12.018
105. Večerić-Haler Ž, Cerar A, Perše M, 2017, (Mesenchymal) Stem Cell-Based Therapy in Cisplatin-Induced Acute Kidney Injury Animal Model: Risk of Immunogenicity and Tumorigenicity. Stem Cells Int, 2017:7304643. https://doi.org/10.1155/2017/7304643
106. Liu Y, Lin L, Zou R, et al., 2018, MSC-derived Exosomes Promote Proliferation and Inhibit Apoptosis of Chondrocytes via lncRNA-KLF3-AS1/miR-206/GIT1 Axis in Osteoarthritis. Cell Cycle, 17:2411–22. https://doi.org/10.1080/15384101.2018.1526603
107. Liang Y, Xu X, Li X, et al., 2020, Chondrocyte-Targeted MicroRNA Delivery by Engineered Exosomes toward a Cell-Free Osteoarthritis Therapy. ACS Appl Mater Interfaces, 12:36938–47. https://doi.org/10.1021/acsami.0c10458