Static Compressive Behavior and Material Failure Mechanism of Trabecular Tantalum Scaffolds Fabricated by Laser Powder Bed Fusion-based Additive Manufacturing
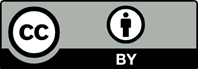
Additively manufactured trabecular tantalum (Ta) scaffolds are promising bone repair materials for load-bearing applications due to their good pore interconnectivity. However, a thorough mechanical behavior evaluation is required before conducting animal studies and clinical research using these scaffolds. In this study, we revealed the compressive mechanical behavior and material failure mechanism of trabecular tantalum scaffolds by compression testing, finite element analysis (FEA), and scanning electron microscopy (SEM). Trabecular tantalum scaffolds with porosities of 65%, 75%, and 85% were fabricated by laser powder bed fusion-based additive manufacturing. Porosity has a significant effect on their compressive mechanical properties. As the porosity decreased from 85% to 65%, the compressive yield strength and elastic modulus increased from 11.9 MPa to 35.7 MPa and 1.1 GPa to 3.0 GPa, respectively. Compression testing results indicate that trabecular tantalum scaffolds demonstrate ductile deformation and excellent mechanical reliability. No macroscopic cracks were found when they were subjected to strain up to 50%. SEM observations showed that material failure results from tantalum strut deformation and fracture. Most microcracks occurred at conjunctions, whereas few of them appear on the struts. FEA-generated compressive stress distribution and material deformation were consistent with experimental results. Stress concentrates at strut conjunctions and vertical struts, where fractures occur during compression testing, indicating that the load-bearing capability of trabecular tantalum scaffolds can be enhanced by strengthening strut conjunctions and vertical struts. Therefore, additively manufactured trabecular tantalum scaffolds can be used in bone tissue reconstruction applications.
1. Matsuno H, Yokoyama A, Watari F, et al., 2001, Biocompatibility and Osteogenesis of Refractory Metal Implants, Titanium, Hafnium, Niobium, Tantalum and Rhenium. Biomaterials, 22:1253–62. https://doi.org/10.1016/S0142-9612(00)00275-1
2. Ruperez E, Manero JM, Riccardi K, et al., 2015, Development of Tantalum Scaffold for Orthopedic Applications Produced by Space-holder Method. Mater Des, 83:112–19. https://doi.org/10.1016/j.matdes.2015.05.067
3. Wang Q, Qiao YQ, Cheng MQ, et al., 2016, Tantalum Implanted Entangled Porous Titanium Promotes Surface Osseointegration and Bone Ingrowth. Sci Rep, 6:13. https://doi.org/10.1038/srep26248
4. Hacking S, Bobyn J, Toh KK, et al., 2000, Fibrous Tissue Ingrowth and Attachment to Porous Tantalum. J Biomed Mater Res, 52:631–8. https://doi.org/10.1002/1097-4636(20001215)52:4<631: AID-JBM7>3.0.CO;2-6
5. Wauthle R, van der Stok J, Yavari SA, et al., 2015, Additively Manufactured Porous Tantalum Implants. Acta Biomater, 14:217–25. https://doi.org/10.1016/j.actbio.2014.12.003
6. Bandyopadhyay A, Mitra I, Shivaram A, et al., 2019, Direct Comparison of Additively Manufactured Porous Titanium and Tantalum Implants Towards In Vivo Osseointegration. Addit Manuf, 28:259–66. https://doi.org/10.1016/j.addma.2019.04.025
7. Wang H, Su KX, Su LZ, et al., 2019, Comparison of 3D-printed Porous Tantalum and Titanium Scaffolds on Osteointegration and Osteogenesis. Mater Sci Eng C Mater Biol Appl, 104:9. https://doi.org/10.1016/j.msec.2019.109908
8. Dou XJ, Wei XW, Liu G, et al., 2019, Effect of Porous Tantalum on Promoting the Osteogenic Differentiation of Bone Marrow Mesenchymal Stem Cells In Vitro through the MAPK/ERK Signal Pathway. J Orthop Transl, 19:81–93. https://doi.org/10.1016/j.jot.2019.03.006
9. Guo Y, Xie K, Jiang WB, et al., 2019, In Vitro and In Vivo Study of 3D-Printed Porous Tantalum Scaffolds for Repairing Bone Defects. ACS Biomater Sci Eng, 5:1123–33. https://doi.org/10.1021/acsbiomaterials.8b01094
10. Van Steenkiste T, Gorkiewicz D, 2004, Analysis of Tantalum Coatings Produced by the Kinetic Spray Process. J Therm Spray Techn, 13:265–73. https://doi.org/10.1361/10599630419418
11. Bobyn JD, Stackpool GJ, Hacking SA, et al., 1999, Characteristics of Bone Ingrowth and Interface Mechanics of a New Porous Tantalum Biomaterial. J Bone Joint Surg Br, 81:907. https://doi.org/10.1302/0301-620X.81B5.9283
12. Jenkins DR, Odland AN, Sierra RJ, et al., 2017, Minimum Five-Year Outcomes with Porous Tantalum Acetabular Cup and Augment Construct in Complex Revision Total Hip Arthroplasty. J Bone Joint Surg Am, 99:7. https://doi.org/10.2106/JBJS.16.00125
13. Huang W, Gong X, Sandiford TE, et al., 2019, Outcome after a New Porous Tantalum Rod Implantation for Treatment of Early-stage Femoral Head Osteonecrosis. Ann Transl Med, 7:11. https://doi.org/10.21037/atm.2019.08.86
14. Peng K, Wang Y, Zhu J, et al., 2020, Repair of Non-traumatic Femoral Head Necrosis by Marrow Core Decompression with Bone Grafting and Porous Tantalum Rod Implantation. Pak J Med Sci, 36:1392. https://doi.org/10.12669/pjms.36.6.2176
15. Zhou YS, Zhu YC, 2013, Three-dimensional Ta Foams Produced by Replication of NaCl Space-holders. Mater Lett, 99:8–10. https://doi.org/10.1016/j.matlet.2013.02.068
16. Wang H, Li QJ, Wang Q, et al., 2017, Enhanced Repair of Segmental Bone Defects in Rabbit Radius by Porous Tantalum Scaffolds Modified with the RGD Peptide. J Mater Sci-Mater Med, 28:10. https://doi.org/10.1007/s10856-017-5860-4
17. Sukumar VR, Golla BR, Shaik MA, et al., 2019, Modeling and Characterization of Porous Tantalum Scaffolds. Trans Indian Inst Met, 72:935–49. https://doi.org/10.1007/s12666-018-01556-1
18. Maconachie T, Leary M, Lozanovski B, et al., 2019, SLM Lattice Structures: Properties, Performance, Applications and Challenges. Mater Des, 183:108137. https://doi.org/10.1016/j.matdes.2019.108137
19. Gong H, Rafi K, Gu H, et al., 2014, Analysis of Defect Generation in Ti-6Al-4V Parts Made Using Powder Bed Fusion Additive Manufacturing Processes. Addit Manuf, 1:87–98. https://doi.org/10.1016/j.addma.2014.08.002
20. Sing SL, Kuo CN, Shih CT, et al., 2021, Perspectives of Using Machine Learning in Laser Powder Bed Fusion for Metal Additive Manufacturing. Virtual Phys Prototy, 16:372–86. https://doi.org/10.1080/17452759.2021.1944229
21. Qin YC, Qi QF, Shi PZ, et al., 2021, Automatic Determination of Part Build Orientation for Laser Powder Bed Fusion. Virtual Phys Prototype, 16:29–49. https://doi.org/10.1080/17452759.2020.1832793
22. Sing SL, Huang S, Goh GD, et al., 2021, Emerging Metallic Systems for Additive Manufacturing: In-Situ Alloying and Multi-metal Processing in Laser Powder Bed Fusion. Prog Mater Sci, 119:100795. https://doi.org/10.1016/j.pmatsci.2021.100795
23. Liu R, Chen Y, Liu Y, et al., 2021, Topological Design of a Trabecular Bone Structure With Morphology and Mechanics Control for Additive Manufacturing. IEEE Access, 9:11123–33. https://doi.org/10.1109/ACCESS.2021.3050745
24. Wang GJ, Shen LD, Zhao JF, et al., 2018, Design and Compressive Behavior of Controllable Irregular Porous Scaffolds: Based on Voronoi-Tessellation and for Additive Manufacturing. ACS Biomater Sci Eng, 4:719–27. https://doi.org/10.1021/acsbiomaterials.7b00916
25. Yang JZ, Jin X, Gao HR, et al., 2020, Additive Manufacturing of Trabecular Tantalum Scaffolds by Laser Powder Bed Fusion: Mechanical Property Evaluation and Porous Structure Characterization. Mater Charact, 170:110694. https://doi.org/10.1016/j.matchar.2020.110694
26. Huang S, Narayan RL, Tan JH, et al., 2021, Resolving the Porosity-unmelted Inclusion Dilemma during In-Situ Alloying of Ti34Nb Via Laser Powder Bed Fusion. Acta Mater, 204:116522. https://doi.org/10.1016/j.actamat.2020.116522
27. Nadammal N, Mishurova T, Fritsch T, et al., 2021, Critical Role of Scan Strategies on the Development of Microstructure, Texture, and Residual Stresses during Laser Powder Bed Fusion Additive Manufacturing. Addit Manuf, 38:101792. https://doi.org/10.1016/j.addma.2020.101792
28. Chen HS, Li QJ, Liu G, 2011, The Development Status and Trend of Environmental Friendly Vacuum Pressure Impregnation Resin at Home and Abroad. Insul Mater, 2. https://doi.org/10.16790/j.cnki.1009-9239.im.2011.02.010
29. Škoro G, Bennett J, Edgecock T, et al., 2011, Dynamic Young’s Moduli of Tungsten and Tantalum at High Temperature and Stress. J Nucl Mater, 409:40–6. https://doi.org/10.1016/j.jnucmat.2010.12.222
30. Shimko DA, Shimko VF, Sander EA, et al., 2005, Effect of Porosity on the Fluid Flow Characteristics and Mechanical Properties of Tantalum Scaffolds. J Biomed Mater Res B, 73:315–24. https://doi.org/10.1002/jbm.b.30229
31. Martens M, Van Audekercke R, Delport P, et al., 1983, The Mechanical Characteristics of Cancellous Bone at the Upper Femoral Region. J Biomech, 16:971–83. https://doi.org/10.1016/0021-9290(83)90098-2
32. Turner CH, Cowin SC, Rho JY, et al., 1990, The Fabric Dependence of the Orthotropic Elastic Constants of Cancellous Bone. J Biomech, 23:549–61. https://doi.org/10.1016/0021-9290(90)90048-8
33. Keaveny TM, Morgan EF, Niebur GL, et al., 2001, Biomechanics of Trabecular Bone. Annu Rev Biomed Eng, 3:307–33.
34. Renders G, Mulder L, Van Ruijven L, et al., 2007, Porosity of Human Mandibular Condylar Bone. J Anat, 210:239–48. https://doi.org/10.1111/j.1469-7580.2007.00693.x
35. Yang E, Leary M, Lozanovski B, et al., 2019, Effect of Geometry on the Mechanical Properties of Ti-6Al-4V Gyroid Structures Fabricated Via SLM: A Numerical Study. Mater Des, 184:108165. https://doi.org/10.1016/j.matdes.2019.108165
36. Ghouse S, Babu S, Nai K, et al., 2018, The Influence of Laser Parameters, Scanning Strategies and Material on the Fatigue Strength of a Stochastic Porous Structure. Addit Manuf, 22:290–301. https://doi.org/10.1016/j.addma.2018.05.024
37. Sing SL, Wiria FE, Yeong WY, 2018, Selective Laser Melting of Lattice Structures: A Statistical Approach to Manufacturability and Mechanical Behavior. Robot Cim Int Manuf, 49:170–180. https://doi.org/10.1016/j.rcim.2017.06.006
38. Huang S, Sing SL, de Looze G, et al., 2020, Laser Powder Bed Fusion of Titanium-tantalum Alloys: Compositions and Designs for Biomedical Applications. J Mech Behav Biomed Mater, 108:103775. https://doi.org/10.1016/j.jmbbm.2020.103775
39. Petite H, Viateau V, Bensaid W, et al., 2000, Tissue-engineered Bone Regeneration. Nat Biotechnol, 18:959–63. https://doi.org/10.1038/79449
40. Hollister SJ, 2005, Porous Scaffold Design for Tissue Engineering. Nat Mater, 4:518–24. https://doi.org/10.1038/nmat1683
41. Chen Z, Yan X, Yin S, et al., 2020, Influence of the Pore Size and Porosity of Selective Laser Melted Ti6Al4V ELI Porous Scaffold on Cell Proliferation, Osteogenesis and Bone Ingrowth. Mat Sci Eng C Mater, 106:110289. https://doi.org/10.1016/j.msec.2019.110289
42. Eichi T, Hiroko T, Hideaki I, et al., 1997, Pore Size of Porous Hydroxyapatite as the Cell-substratum Controls BMP induced Osteogenesis. J Biochem, 121:317–24. https://doi.org/10.1093/oxfordjournals.jbchem.a021589
43. Karageorgiou V, Kaplan D, 2005, Porosity of 3D Biomaterial Scaffolds and Osteogenesis. Biomaterials, 26:5474–91. https://doi.org/10.1016/j.biomaterials.2005.02.002
44. Niinomi M, Nakai M, 2011, Titanium-based Biomaterials for Preventing Stress Shielding Between Implant Devices and Bone. Int J Biomater, 2011:836587. https://doi.org/10.1155/2011/836587
45. Ahmadi S, Campoli G, Yavari SA, et al., 2014, Mechanical Behavior of Regular Open-cell Porous Biomaterials Made of Diamond Lattice Unit Cells. J Mech Behav Biomed Mater, 34:106–15. https://doi.org/10.1016/j.jmbbm.2014.02.003
46. Li ZH, Nie YF, Liu B, et al., 2020, Mechanical Properties of AlSi10Mg Lattice Structures Fabricated by Selective Laser Melting. Mater Des, 192:108709. https://doi.org/10.1016/j.matdes.2020.108709
47. Petit C, Maire E, Meille S, et al., 2016, CoCrMo Cellular Structures Made by Electron Beam Melting Studied by Local Tomography and Finite Element Modelling. Mater Charact, 116:48–54. https://doi.org/10.1016/j.matchar.2016.04.006
48. Yang A, Wen C, Hodgson PD, et al., 2012, Investigation of Cell Shape Effect on the Mechanical Behaviour of Open-cell Metal Foams. Comp Mater Sci, 55:1–9. https://doi.org/10.1016/j.commatsci.2011.11.030
49. Li S, Xu Q, Wang Z, et al., 2014, Influence of Cell Shape on Mechanical Properties of Ti-6Al-4V Meshes Fabricated by Electron Beam Melting Method. Acta Biomater, 10:4537–47. https://doi.org/10.1016/j.actbio.2014.06.010
50. Zhang MK, Yang YQ, Wang D, et al., 2019, Microstructure and Mechanical Properties of CuSn/18Ni300 Bimetallic Porous Structures Manufactured by Selective Laser Melting. Mater Des, 165:107583. https://doi.org/10.1016/j.matdes.2019.107583
51. Cosma C, Drstvensek I, Berce P, et al., 2020, Physical-Mechanical Characteristics and Microstructure of Ti6Al7Nb Lattice Structures Manufactured by Selective Laser Melting. Materials, 13:4123. https://doi.org/10.3390/ma13184123
52. Shin J, Kim S, Jeong D, et al., 2012, Finite Element Analysis of Schwarz P Surface Pore Geometries for Tissue-engineered Scaffolds. Math Probl Eng, 2012:865–83. https://doi.org/10.1155/2012/694194
53. Soro N, Brassart L, Chen Y, et al., 2018, Finite Element Analysis of Porous Commercially Pure Titanium for Biomedical Implant Application. Mat Sci Eng A Struct, 725:43–50. https://doi.org/10.1016/j.msea.2018.04.009
54. Zhang B, Pei X, Zhou C, et al., 2018, The Biomimetic Design and 3D Printing of Customized Mechanical Properties Porous Ti6Al4V Scaffold for Load-bearing Bone Reconstruction. Mater Des, 152:30–9. https://doi.org/10.1016/j.matdes.2018.04.065
55. Du Y, Liang H, Xie D, et al., 2019, Finite Element Analysis of Mechanical Behavior, Permeability of Irregular Porous Scaffolds and Lattice-based Porous Scaffolds. Mater Res Express, 6:105407. https://doi.org/10.1088/2053-1591/ab3ac1
56. Patel R, Lu M, Diermann SH, et al., 2019, Deformation Behavior of Porous PHBV Scaffold in Compression: A Finite Element Analysis Study. J Mech Behav Biomed Mater, 96:1–8. https://doi.org/10.1016/j.jmbbm.2019.04.030
57. Peng WM, Liu YF, Jiang XF, et al., 2019, Bionic Mechanical Design and 3D Printing of Novel Porous Ti6Al4V Implants for Biomedical Applications. J Zhejiang Univ Sci B, 20:647–59. https://doi.org/10.1631/jzus.B1800622
58. Yang L, Yan C, Cao W, et al., 2019, Compression-Compression Fatigue Behaviour of Gyroid-type Triply Periodic Minimal Surface Porous Structures Fabricated by Selective Laser Melting. Acta Mater, 181:49–66. https://doi.org/10.1016/j.actamat.2019.09.042
59. Zhao L, Pei X, Jiang L, et al., 2019, Bionic Design and 3D Printing of Porous Titanium Alloy Scaffolds for Bone Tissue Repair. Compos Part B Eng, 162:154–61. https://doi.org/10.1016/j.compositesb.2018.10.094