Fabrication and Biomedical Applications of Heart-on-a-chip
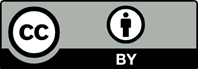
Heart diseases have become the main killer threatening human health, and various methods have been developed to study heart disease. Among them, heart-on-a-chip has emerged in recent years as a method for constructing disease (or normal) models in vitro and is considered as a promising tool to study heart diseases. Compared with other methods, the advantages of heart-on-a-chip include the high portability, high throughput, and the capability to mimic microenvironments in vivo. It has shown a great potential in disease mechanism study and drug screening. In this paper, we review the recent advances in hearton-a-chip, including the fabrication methods (e.g., 3D bioprinting) and biomedical applications. By analyzing the structure of the existing heart-on-a-chip, we proposed that a highly integrated heart-on-a-chip includes four elements: Microfluidic chips, cells/microtissues, microactuators to construct the microenvironment, and microsensors for results readout. Finally, the current challenges and future directions of heart-on-a-chip are discussed.
1. Yusuf SW, Cipolla C, Durand JB, et al., 2011, Cancer and Cardiovascular Disease. Cardiol Res Pract, 2011:943748.
2. Benjamin EJ, Muntner P, Alonso A, et al., 2020, Heart Disease and Stroke Statistics-2019 Update: A Report from the American Heart Association. Circulation, 141:E33. https://doi.org/10.1161/cir.0000000000000746
3. Denayer T, StöHr T, Roy MT, 2014, Animal Models in Translational Medicine: Validation and Prediction. New Horiz Transl Med, 2:5–11.
4. Chi CW, Lao YH, Ahmed AH, et al., 2020, High-Throughput Tumor-on-a-Chip Platform to Study Tumor-Stroma Interactions and Drug Pharmacokinetics. Adv Healthc Mater, 9:2000880. https://doi.org/10.1002/adhm.202000880
5. Lu HF, Leong MF, Lim TC, et al., 2017, Engineering a Functional Three-dimensional Human Cardiac Tissue Model for Drug Toxicity Screening. Biofabrication, 9:025011. https://doi.org/10.1088/1758-5090/aa6c3a
6. Zhang Y, Aleman J, Arneri A, et al., 2015, From Cardiac Tissue Engineering to Heart-on-a-chip: Beating Challenges. Biomed Mater, 10:034006. https://doi.org/10.1088/1748-6041/10/3/034006
7. Wei X, Zhuang L, Li H, et al., 2020, Advances in Multidimensional Cardiac Biosensing Technologies: From Electrophysiology to Mechanical Motion and Contractile Force. Small, 16:2005828. https://doi.org/10.1002/smll.202005828
8. Huang S, Yang Y, Qi Y, et al., 2018, Engineered Circulatory Scaffolds for Building Cardiac Tissue. J Thorac Dis, 10:S2312–28. https://doi.org/10.21037/jtd.2017.12.92
9. Zhao Y, Rafatian N, Wang E, et al., 2020, Towards Chamber Specific Heart-on-a-chip for Drug Testing Applications. Adv Drug Deliv Rev, 165–166:60–76. https://doi.org/10.1016/j.addr.2019.12.002
10. Guerzoni L, Tsukamoto Y, Gehlen DB, et al., 2019, A Layer-by-Layer Single-Cell Coating Technique To Produce Injectable Beating Mini Heart Tissues via Microfluidics. Biomacromolecules, 20:3746–54. https://doi.org/10.1021/acs.biomac.9b00786
11. Derda R, Laromaine A, Mannoto A, et al., 2009, Paper supported 3D Cell Culture for Tissue-based Bioassays. Proc Natl Acad Sci U S A, 106:18457–62.
12. Lind JU, Busbee TA, Valentine AD, et al., 2017, Instrumented Cardiac Microphysiological Devices via Multimaterial Three-dimensional Printing. Nat Mater, 16:303. https://doi.org/10.1038/nmat4782
13. Kim BS, Jang J, Chae S, et al., 2016, Three-dimensional Bioprinting of Cell-laden Constructs with Polycaprolactone Protective Layers for Using Various Thermoplastic Polymers. Biofabrication, 8:035013. https://doi.org/10.1088/1758-5090/8/3/035013
14. Yi HG, Choi YJ, Kang KS, et al., 2016, A 3D-printed Local Drug Delivery Patch for Pancreatic Cancer Growth Suppression. J Control Release, 238:231–41. https://doi.org/10.1016/j.jconrel.2016.06.015
15. Kolesky DB, TRuby RL, Gladman AS, et al., 2014, 3D Bioprinting of Vascularized, Heterogeneous Cell-laden Tissue Constructs. Adv Mater, 26:3124–30. https://doi.org/10.1002/adma.201305506
16. Yashiro M, Oouchi T, Tsushima H, et al., 2017, Excimer Laser Gas Usage Reduction Technology for Semiconductor Manufacturing, SPIE Adv Lithogr, 10147:1014710. https://doi.org/10.1117/12.2257972
17. Randall JN, Owen JH, Lake J, et al., 2019, Next Generation of Extreme-Resolution Electron Beam Lithography. J Vac Sci Technol, 37:061605. https://doi.org/10.1116/1.5119392
18. He Y, Wu Y, Fu JZ, et al., 2016, Developments of 3D Printing Microfluidics and Applications in Chemistry and Biology: A Review. Electroanalysis, 28:1658–78. https://doi.org/10.1002/elan.201600043
19. He Y, Qiu J, Fu J, et al., 2015, Printing 3D Microfluidic Chips with a 3D SUGAR Printer. Microfluid Nanofluidics, 19:447–56. https://doi.org/10.1007/s10404-015-1571-7
20. Caulfield JB, Borg TK, 1979, The Collagen Network of the Heart. Lab Invest, 40:364–72.
21. Ramme AP, Koenig L, Hasenberg T, et al., 2018, Towards an Autologous iPSC-Derived Patient-on-a-Chip.
22. Grevesse T, Verseavel M, Circelli G, et al., 2013, A Simple Route to Functionalize Polyacrylamide Hydrogels for the Independent Tuning of Mechanotransduction Cues. Lab Chip, 13:777–80. https://doi.org/10.1039/c2lc41168g
23. Cimetta E, Pizzato S, Bollini S, et al., 2009, Production of Arrays of Cardiac and Skeletal Muscle Myofibers by Micropatterning Techniques on a Soft Substrate. Biomed Microdevices, 11:389–400. https://doi.org/10.1007/s10544-008-9245-9
24. Camelliti P, Gallagher JO, Kohl P, et al., 2006, Micropatterned Cell Cultures on Elastic Membranes as an In Vitro Model of Myocardium. Nat Protoc, 1:1379–91. https://doi.org/10.1038/nprot.2006.203
25. Annabi N, Tsang K, Mithieux SM, et al., 2013, Highly Elastic Micropatterned Hydrogel for Engineering Functional Cardiac Tissue. Adv Funct Mater, 23:4949–59. https://doi.org/10.1002/adfm.201300570
26. Versaevel M, Grevesse T, Gabriele S, 2012, Spatial Coordination Between Cell and Nuclear Shape within Micropatterned Endothelial Cells. Nat Commun, 3:671. https://doi.org/10.1038/ncomms1668
27. Mccain ML, Agarwal A, Nesmith HW, et al., 2014, Micromolded Gelatin Hydrogels for Extended Culture of Engineered Cardiac Tissues. Biomaterials, 35:5462–71. https://doi.org/10.1016/j.biomaterials.2014.03.052
28. Liu J, Miller K, Ma X, et al., 2020, Direct 3D Bioprinting of Cardiac Micro-Tissues Mimicking Native Myocardium. Biomaterials, 256:120204. https://doi.org/10.1016/j.biomaterials.2020.120204
29. Skardal A, Murphy SV, Devarasetty M, et al., 2017, Multi- Tissue Interactions in an Integrated Three-Tissue Organ-ona-Chip Platform. Sci Rep, 7:8837.
30. Zhang YS, Arneri A, Bersini S, et al., 2016, Bioprinting 3D Microfibrous Scaffolds for Engineering Endothelialized Myocardium and Heart-on-a-Chip. Biomaterials, 110:45–59. https://doi.org/10.1016/j.biomaterials.2016.09.003
31. Colosi C, Shin SR, Manoharan V, et al., 2016, Microfluidic Bioprinting of Heterogeneous 3D Tissue Constructs Using Low-Viscosity Bioink. Adv Mater, 28:677–84. https://doi.org/10.1002/adma.201503310
32. Morgan KY, Sklaviadis D, Tochka ZL, et al., 2016, Multi-Material Tissue Engineering Scaffold with Hierarchical Pore Architecture. Adv Funct Mater, 26:5873–83. https://doi.org/10.1002/adfm.201601146
33. Miri AK, Daniel N, Luis I, et al., 2018, Microfluidics-Enabled Multimaterial Maskless Stereolithographic Bioprinting. Adv Mater, 30:1800242. https://doi.org/10.1002/adma.201800242
34. Fenech M, Girod V, Claveria V, et al., 2019, Microfluidic Blood Vasculature Replicas using Backside Lithography. Lab Chip, 19:2096106. https://doi.org/10.1039/c9lc00254e
35. Bartholomaand P, Gorjup E, Monz D, et al., 2005, Three-Dimensional In Vitro Reaggregates of Embryonic Cardiomyocytes: A Potential Model System for Monitoring Effects of Bioactive Agents. J Biomol Screen, 10:814–22. https://doi.org/10.1177/1087057105280070
36. Ronaldson-Bouchard K, Ma SP, Yeager K, et al., 2018, Advanced Maturation of Human Cardiac Tissue Grown from Pluripotent Stem Cells. Nature, 556:239–43. https://doi.org/10.1038/s41586-018-0016-3
37. Tandon N, Marsano A, Maidhof R, et al., 2011, Optimization of Electrical Stimulation Parameters for Cardiac Tissue Engineering. J Tissue Eng Regen Med, 5:E115–25. https://doi.org/10.1002/term.377
38. Valls-Margarit M, Iglesias-Garcia O, di Guglielmo C, et al., 2019, Engineered Macroscale Cardiac Constructs Elicit Human Myocardial Tissue-like Functionality. Stem Cell Reports, 13:207–20. https://doi.org/10.1016/j.stemcr.2019.05.024
39. Zhang N, Stauffer F, Simona BR, et al., 2018, Multifunctional 3D Electrode Platform for Real-Time In Situ Monitoring and Stimulation of Cardiac Tissues. Biosens Bioelectron, 112:149–55. https://doi.org/10.1016/j.bios.2018.04.037
40. Moon SH, Cho YW, Shim HE, et al., 2020, Electrically Stimulable Indium Tin Oxide Plate for Long-Term In Vitro Cardiomyocyte Culture. Biomater Res, 24:10. https://doi.org/10.21203/rs.3.rs-22137/v2
41. Oyunbaatar NE, Shanmugasundaram A, Jeong YJ, et al., 2020, Micro-Patterned SU-8 Cantilever Integrated with Metal Electrode for Enhanced Electromechanical Stimulation of Cardiac Cells. Colloids Surf B Biointerfaces, 186:110682. https://doi.org/10.1016/j.colsurfb.2019.110682
42. Yuk H, Lu B, Lin S, et al., 2020, 3D Printing of Conducting Polymers. Nat Commun, 11:1604.
43. Adly N, Weidlich S, Seyock S, et al., 2018, Printed Microelectrode Arrays on Soft Materials: From PDMS to Hydrogels. NPJ Flex Electron, 2:15. https://doi.org/10.1038/s41528-018-0027-z
44. Zhao G, Zhang X, Li B, et al., 2020, Solvent-Free Fabrication of Carbon Nanotube/Silk Fibroin Electrospun Matrices for Enhancing Cardiomyocyte Functionalities. ACS Biomater Sci Eng, 6:1630–40. https://doi.org/10.1021/acsbiomaterials.9b01682
45. Stoppel WL, Kaplan DL, Black LD, 2016, Electrical and Mechanical Stimulation of Cardiac Cells and Tissue Constructs. Adv Drug Deliv Rev, 96:135–55. https://doi.org/10.1016/j.addr.2015.07.009
46. Mihic A, Li J, Miyagi Y, et al., 2014, The Effect of Cyclic Stretch on Maturation and 3D Tissue Formation of Human Embryonic Stem Cell-Derived Cardiomyocytes. Biomaterials, 35:2798–808. https://doi.org/10.1016/j.biomaterials.2013.12.052
47. Marsano A, Conficconi C, Lemme M, et al., 2016, Beating Heart on a Chip: A Novel Microfluidic Platform to Generate Functional 3D Cardiac Microtissues. Lab Chip, 16:599–610. https://doi.org/10.1039/c5lc01356a
48. Chinali M, Simone GD, Roman MJ, et al., 2005, Left Atrial Systolic Force and Cardiovascular Outcome. The Strong Heart Study. Am J Hypertens, 18:1570–6.
49. Bajaj P, Tang X, Saif TA, et al., 2010, Stiffness of the Substrate Influences the Phenotype of Embryonic Chicken Cardiac Myocytes. J Biomed Mater Res Part A, 95A:1261–9. https://doi.org/10.1002/jbm.a.32951
50. Hong SP, Song S, Cho SW, et al., 2017, Generation of PDGFRα+ Cardioblasts from Pluripotent Stem Cells. Sci Rep, 7:41840.
51. Ruan JL, Tulloch NL, Razumova MV, et al., 2016, Mechanical Stress Conditioning and Electrical Stimulation Promote Contractility and Force Maturation of Induced Pluripotent Stem Cell-Derived Human Cardiac Tissue. Circulation, 134:1557–67. https://doi.org/10.1161/circulationaha.114.014998
52. Visone R, Talò G, Occhetta P, et al., 2018, A Microscale Biomimetic Platform for Generation and Electro-Mechanical Stimulation of 3D Cardiac Microtissues. APL Bioeng, 2:046102. https://doi.org/10.1063/1.5037968
53. Cho KW, Lee WH, Kim BS, et al., 2020, Sensors in Heart-on a-Chip: A Review on Recent Progress. Talanta, 219:121269. https://doi.org/10.1016/j.talanta.2020.121269
54. Plotnikov SV, Sabass B, Schwarz U, et al., 2014, High-Resolution Traction Force Microscopy. Methods Cell Biol, 123:367–94. https://doi.org/10.1016/b978-0-12-420138-5.00020-3
55. Polacheck W, William J, Chen CS, 2016, Measuring Cell-Generated Forces: A Guide to the Available Tools. Nat Methods, 13:415–23. https://doi.org/10.1038/nmeth.3834
56. Yu Y, Fu F, Shang L, et al., 2017, Bioinspired Helical Microfibers from Microfluidics. Adv Mater, 29:1605765. https://doi.org/10.1002/adma.201605765
57. Ma X, Dewan S, Liu J, et al., 2018, 3D Printed Micro-Scale Force Gauge Arrays to Improve Human Cardiac Tissue Maturation and Enable High Throughput Drug Testing. Acta Biomat, 95:319–27. https://doi.org/10.1016/j.actbio.2018.12.026
58. Tan JL, Tien J, Pirone DM, et al., 2003, Cells Lying on a Bed of Microneedles: An Approach to Isolate Mechanical Force. Proc Natl Acad Sci U S A, 100:1484–9. https://doi.org/10.1073/pnas.0235407100
59. Agarwal A, Goss JA, Cho A, et al., 2013, Microfluidic Heart on a Chip for Higher Throughput Pharmacological Studies. Lab Chip, 13:3599–608. https://doi.org/10.1039/c3lc50350j
60. Oyunbaatar NE, Shanmugasundaram A, Lee DW, 2019, Contractile Behaviors of Cardiac Muscle Cells on Mushroom-Shaped Micropillar Arrays. Colloids Surf B Biointerfaces, 174:103–9. https://doi.org/10.1016/j.colsurfb.2018.10.058
61. Vandenburgh H, Shansky J, Benesch-Lee F, et al., 2008, Drug-Screening Platform Based on the Contractility of Tissue-Engineered Muscle. Muscle Nerve, 37:438–47. https://doi.org/10.1002/mus.20931
62. Zhao Y, Wang EY, Davenport LH, et al., 2019, A Multimaterial Microphysiological Platform Enabled by Rapid Casting of Elastic Microwires. Adv Healthc Mater, 8:1801187. https://doi.org/10.1002/adhm.201801187
63. Mastikhina O, Moon BU, Williams K, et al., 2020, Human Cardiac Fibrosis-on-a-Chip Model Recapitulates Disease Hallmarks and Can Serve as a Platform for Drug Testing. Biomaterials, 233:119741. https://doi.org/10.1016/j.biomaterials.2019.119741
64. Chan V, Jeong JH, Bajaj P, et al., 2011, Multi-Material Bio-Fabrication of Hydrogel Cantilevers and Actuators with Stereolithography. Lab Chip, 12:88–98. https://doi.org/10.1039/c1lc20688e
65. Fu F, Shang L, Chen Z, et al., 2018, Bioinspired Living Structural Color Hydrogels. Sci Robot, 3:aar8580.
66. Sun L, Chen Z, Bian F, et al., 2020, Bioinspired Soft Robotic Caterpillar with Cardiomyocyte Drivers. Adv Funct Mater, 30:1907820. https://doi.org/10.1002/adfm.201907820
67. Kim DS, Jeong YJ, Lee BK, et al., 2017, Piezoresistive Sensor-Integrated PDMS Cantilever: A New Class of Device for Measuring the Drug-Induced Changes in the Mechanical Activity of Cardiomyocytes. Sens Actuators B Chem, 240:566–72. https://doi.org/10.1016/j.snb.2016.08.167
68. Wang L, Dou W, Malhi M, et al., 2018, Microdevice Platform for Continuous Measurement of Contractility, Beating Rate, and Beating Rhythm of Human-Induced Pluripotent Stem Cell-Cardiomyocytes inside a Controlled Incubator Environment. Acs Appl Mater Interfaces, 10:21173–83. https://doi.org/10.1021/acsami.8b05407.s001
69. Coln EA, Colon A, Long CJ, et al., 2019, Piezoelectric bioMEMS Cantilever for Measurement of Muscle Contraction and for Actuation of Mechanosensitive Cells. MRS Commun, 9:1186–92. https://doi.org/10.1557/mrc.2019.129
70. Wang A, Liu Z, Hu M, et al., 2018, Piezoelectric Nanofibrous Scaffolds as In Vivo Energy Harvesters for Modifying Fibroblast Alignment and Proliferation in Wound Healing. Nano Energy, 43:63–71. https://doi.org/10.1016/j.nanoen.2017.11.023
71. Sakamiya M, Fang Y, Mo X, et al., 2020, A Heart-on-a-Chip Platform for Online Monitoring of Contractile Behavior Via Digital Image Processing and Piezoelectric Sensing Technique. Med Eng Phys, 75:36–44. https://doi.org/10.1016/j.medengphy.2019.10.001
72. Lind JU, Yadid M, Perkins I, et al., 2017, Cardiac Microphysiological Devices with Flexible Thin-Film Sensors for Higher-Throughput Drug Screening. Lab Chip, 17: 3692–703. https://doi.org/10.1039/c7lc00740j
73. Kang D, Pikhitsa PV, Choi YW, et al., 2014, Ultrasensitive Mechanical Crack-Based Sensor Inspired by the Spider Sensory System. Nature, 516:222–6. https://doi.org/10.1038/nature14002
74. Kim DS, Choi YW, Shanmugasundaram A, et al., 2020, Highly Durable Crack Sensor Integrated with Silicone Rubber Cantilever for Measuring Cardiac Contractility. Nat Commun, 11:535. https://doi.org/10.1038/s41467-019-14019-y
75. Kireev D, Seyock S, Lewen J, et al., 2017, Graphene Multielectrode Arrays as a Versatile Tool for Extracellular Measurements. Adv Healthc Mater, 6:1601433. https://doi.org/10.1002/adhm.201601433
76. Qian F, Huang C, Lin YD, et al., 2017, Simultaneous Electrical Recording of Cardiac Electrophysiology and Contraction on Chip. Lab Chip, 17:1732–9. https://doi.org/10.1039/c7lc00210f
77. Tian B, Cohen-Karni T, Qing Q, et al., 2010, Three-Dimensional, Flexible Nanoscale Field-Effect Transistors as Localized Bioprobes. Science, 329:830–4. https://doi.org/10.1126/science.1192033
78. Abbott J, Ye T, Qin L, et al., 2017, CMOS Nanoelectrode Array for All-Electrical Intracellular Electrophysiological Imaging. Nat Nanotechnol, 12:460–6. https://doi.org/10.1038/nnano.2017.3
79. Dai X, Zhou W, Gao T, et al., 2016, Three-Dimensional Mapping and Regulation of Action Potential Propagation in Nanoelectronics-Innervated Tissues. Nat Nanotechnol, 11:776–82. https://doi.org/10.1038/nnano.2016.96
80. Kaneko T, Kojima K, Yasuda K, 2007, An On-Chip Cardiomyocyte Cell Network Assay for Stable Drug Screening Regarding Community Effect of Cell Network Size. Analyst, 132:892–8. https://doi.org/10.1039/b704961g
81. Aung A, Bhullar IS, Theprungsirikul J, et al., 2016, 3D Cardiac Mu Tissues Within a Microfluidic Device with Real-Time Contractile Stress Readout. Lab Chip, 16:153–62. https://doi.org/10.1039/c5lc00820d
82. Mai-Dung N, Tinney JP, Yuan F, et al., 2013, Cardiac Cell Culture Model As a Left Ventricle Mimic for Cardiac Tissue Generation. Anal Chem, 85:8773–9. https://doi.org/10.1021/ac401910d
83. Chen Y, Chan HN, Michael SA, et al., 2017, A Microfluidic Circulatory System Integrated with Capillary-Assisted Pressure Sensors. Lab Chip, 17:653–62. https://doi.org/10.1039/c6lc01427e
84. Savoji H, Mohammadi MH, Rafatian N, et al., 2019, Cardiovascular Disease Models: A Game Changing Paradigm in Drug Discovery and Screening. Biomaterials, 198:3–26. https://doi.org/10.1016/j.biomaterials.2018.09.036
85. Ren L, Liu W, Wang Y, et al., 2013, Investigation of Hypoxia-Induced Myocardial Injury Dynamics in a Tissue Interface Mimicking Microfluidic Device. Anal Chem, 85:235–44. https://doi.org/10.1021/ac3025812
86. Liu H, Bolonduro OA, Hu N, et al., 2020, Heart-on-a-Chip Model with Integrated Extra-and Intracellular Bioelectronics for Monitoring Cardiac Electrophysiology under Acute Hypoxia. Nano Lett, 20:2585–93. https://doi.org/10.1021/acs.nanolett.0c00076
87. Martewicz S, Michielin F, Serena E, et al., 2012, Reversible Alteration of Calcium Dynamics in Cardiomyocytes during Acute Hypoxia Transient in a Microfluidic Platform. Integr Biol, 4:153–64. https://doi.org/10.1039/c1ib00087j
88. Sadeghi AH, Shin SR, Deddens JC, et al., 2017, Engineered 3D Cardiac Fibrotic Tissue to Study Fibrotic Remodeling. Adv Healthc Mater, 6:1601434. https://doi.org/10.1002/adhm.201601434
89. Tse G, 2016, Mechanisms of Cardiac Arrhythmias. J Arrhythm, 32:75–81.
90. Ma Z, Koo S, Finnegan MA, et al., 2014, Three-Dimensional Filamentous Human Diseased Cardiac Tissue Model. Biomaterials, 35:1367–77. https://doi.org/10.1016/j.biomaterials.2013.10.052
91. Brandão KO, Tabel VA, Atsma DE, et al., 2017, Human Pluripotent Stem Cell Models of Cardiac Disease: From Mechanisms to Therapies. Dis Model Mech, 10:1039–59. https://doi.org/10.1242/dmm.030320
92. Ren L, Zhou X, Nasiri R, et al., 2020, Combined Effects of Electric Stimulation and Microgrooves in Cardiac Tissue-ona-Chip for Drug Screening. Small Methods, 4:2000438. https://doi.org/10.1002/smtd.202000438
93. Zhao Y, Rafatian N, Feric NT, et al., 2019, A Platform for Generation of Chamber-Specific Cardiac Tissues and Disease Modeling. Cell, 176:913–27.e18.
94. Huang G, Li F, Zhao X, et al., 2017, Functional and Biomimetic Materials for Engineering of the Three-Dimensional Cell Microenvironment. Chem Rev, 117:12764–850.
95. Yu F, Choudhury D, 2019, Microfluidic Bioprinting for Organ-on-a-Chip Models. Drug Discov Today, 24:1248–57. https://doi.org/10.1016/j.drudis.2019.03.025
96. Gao B, Yang Q, Zhao X, et al., 2016, 4D Bioprinting for Biomedical Applications. Trends Biotechnol, 34:746–56.
97. Shi M, Ling K, Yong KW, et al., 2015, High-Throughput Non-Contact Vitrification of Cell-Laden Droplets Based on Cell Printing. Sci Rep, 5:17928. https://doi.org/10.1038/srep17928
98. Bertassoni LE, Cecconi M, Manoharan V, et al., 2014, Hydrogel Bioprinted Microchannel Networks for Vascularization of Tissue Engineering Constructs. Lab Chip, 14:2202–11. https://doi.org/10.1039/c4lc00030g