Applications of 3D Bioprinted-Induced Pluripotent Stem Cells in Healthcare
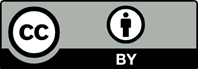
Induced pluripotent stem cell (iPSC) technology and advancements in three-dimensional (3D) bioprinting technology enable scientists to reprogram somatic cells to iPSCs and 3D print iPSC-derived organ constructs with native tissue architecture and function. iPSCs and iPSC-derived cells suspended in hydrogels (bioinks) allow to print tissues and organs for downstream medical applications. The bioprinted human tissues and organs are extremely valuable in regenerative medicine as bioprinting of autologous iPSC-derived organs eliminates the risk of immune rejection with organ transplants. Disease modeling and drug screening in bioprinted human tissues will give more precise information on disease mechanisms, drug efficacy, and drug toxicity than experimenting on animal models. Bioprinted iPSC-derived cancer tissues will aid in the study of early cancer development and precision oncology to discover patient-specific drugs. In this review, we present a brief summary of the combined use of two powerful technologies, iPSC technology, and 3D bioprinting in health-care applications.
1. Takahashi K, Yamanaka S, 2006, Induction of Pluripotent Stem Cells from Mouse Embryonic and Adult Fibroblast Cultures by Defined Factors. Cell, 126:663–76. DOI: 10.1016/j.cell.2006.07.024.
2. Yu J, Vodyanik MA, Smuga-Otto K, et al., 2007, Thomson, Induced Pluripotent Stem Cell Lines Derived from Human Somatic Cells. Science, 318:1917–20. DOI: 10.1126/science.1151526.
3. Zhang Y, Chen K, Sloan SA, et al., 2004, An RNA-sequencing Transcriptome and Splicing Database of Glia, Neurons, and Vascular Cells of the Cerebral Cortex. J Neurosci, 34:11929–47. DOI: 10.1523/jneurosci.1860-14.2014.
4. Chappell J, Dalton S, 2013, Roles for MYC in the Establishment and Maintenance of Pluripotency. Cold Spring Harb. Perspect Med, 3:a014381. DOI: 10.1101/cshperspect.a014381.
5. Zhang P, Zhang X, Brown J, et al., 2010, Global Healthcare Expenditure on Diabetes for 2010 and 2030. Diabetes Res Clin Pract, 87:293–301. DOI: 10.1016/j.diabres.2010.12.025.
6. Han JW, Yoon YS, 2011, Induced Pluripotent Stem Cells: Emerging Techniques for Nuclear Reprogramming. Antioxidants Redox Signal, 15:1799–820. DOI: 10.1089/ars.2010.3814.
7. An Z, Liu P, Zheng J, et al., 2019, Sox2 and Klf4 as the Functional Core in Pluripotency Induction without Exogenous Oct4. Cell Rep, 29:1986–2000. DOI: 10.1016/j.celrep.2019.10.026.
8. Chen IP, Fukuda K, Fusaki N, et al., 2013, Induced Pluripotent Stem Cell Reprogramming by Integration-free Sendai Virus Vectors from Peripheral Blood of Patients with Craniometaphyseal Dysplasia. Cell Reprogram, 15:503–13. DOI: 10.1089/cell.2013.0037.
9. Sridharan R, Tchieu J, Mason MJ, et al., 2009, Role of the Murine Reprogramming Factors in the Induction of Pluripotency. Cell, 136:364–77. DOI: 10.1016/j.cell.2009.01.001.
10. Kim JB, Greber B, Arazo-Bravo MJ, et al., 2009, Direct Reprogramming of Human Neural Stem Cells by OCT4. Nature, 461:649–53. DOI: 10.1038/nature08436.
11. Stadtfeld M, Hochedlinger K, 2010, Induced Pluripotency: History, Mechanisms, and Applications. Genes Dev, 24:2239–63. DOI: 10.1101/gad.1963910.
12. Yee JK, 2010, Turning Somatic Cells into Pluripotent Stem Cells. Nat Educ, 3:25.
13. Mertens J, Reid D, Lau S, et al., 2018, Aging in a Dish: iPSC-Derived and Directly Induced Neurons for Studying Brain Aging and Age-Related Neurodegenerative Diseases. Annu Rev Genet, 52:271–93. DOI: 10.1146/annurevgenet-120417-031534.
14. Penney J, Ralvenius WT, Tsai LH, 2019, Modeling Alzheimer’s Disease with iPSC-Derived Brain Cells. Mol Psychiatry, 25:148–67. DOI: 10.1038/s41380-019-0468-3.
15. Chen IY, Matsa E, Wu JC, 2016, Induced Pluripotent Stem Cells: At the Heart of Cardiovascular Precision Medicine. Nat Rev Cardiol, 13:333–49. DOI: 10.1038/nrcardio.2016.36.
16. Koch L, Deiwick A, Franke A, et al., 2018, Laser Bioprinting of Human Induced Pluripotent Stem Cells the Effect of Printing and Biomaterials on Cell Survival, Pluripotency, and Differentiation. Biofabrication, 10:035005. DOI: 10.1088/1758-5090/aab981.
17. Maiullari F, Costantini M, Milan M, et al., 2018, A Multicellular 3D Bioprinting Approach for Vascularized Heart Tissue Engineering Based on HUVECs and iPSC-Derived Cardiomyocytes. Sci Rep, 8:13532. DOI: 10.1038/s41598-018-31848-x.
18. Yeung E, Fukunishi T, Bai Y, et al., 2019, Cardiac Regeneration Using Human-induced Pluripotent Stem Cell derived Biomaterial-free 3D-bioprinted Cardiac Patch In Vivo. J Tissue Eng Regen Med, 13:2031-9. DOI: 10.1002/term.2954.
19. Faulkner-Jones A, Fyfe C, Cornelissen DJ, et al., 2015, Bioprinting of Human Pluripotent Stem Cells and their Directed Differentiation into Hepatocyte-like Cells for the Generation of Mini-livers in 3D. Biofabrication, 7:044102. doi.org/10.1088/1758-5090/7/4/044102.
20. Nguyen HX, Hooshmand MJ, Saiwai H, et al., 2017, Systemic Neutrophil Depletion Modulates the Migration and Fate of Transplanted Human Neural Stem Cells to Rescue Functional Repair. J Neurosci, 37:9269–87. DOI: 10.1523/jneurosci.2785-16.2017.
21. Li Y, Lu B, Sheng L, et al., 2018, Hexokinase 2-dependent Hyperglycolysis Driving Microglial Activation Contributes to Ischemic Brain Injury. J Neurochem, 144:186–200. DOI: 10.1111/jnc.14267.
22. Koch SC, Acton D, Goulding M, 2018, Spinal Circuits for Touch, Pain, and Itch. Annu Rev Physiol, 80:189–217. DOI: 10.1146/annurev-physiol-022516-034303.
23. Gungor-Ozkerim PS, Inci I, Zhang YS, et al., 2018, Bioinks for 3D Bioprinting: An Overview. Biomater Sci, 6:915–46. DOI: 10.1039/c7bm00765e.
24. Montero FE, Rezende RA, da Silva JV, et al., 2019, Development of a Smart Bioink for Bioprinting Applications. Front Mech Eng, 5:56. DOI: 10.3389/fmech.2019.00056.
25. Noor N, Shapira A, Edri R, et al., 2019, 3D Printing of Personalized Thick and Perfusable Cardiac Patches and Hearts. Adv Sci, 6:3–10. DOI: 10.1002/advs.201970066.
26. Gopinathan J, Noh I, 2018, Recent Trends in Bioinks for 3D Printing. Biomater Res, 22:11. DOI: 110.1186/s40824-018-0122-1.
27. Gu J, Bradbury J, Xiong C, et al., 2018, Non-autoregressive Neural Machine Translation. 6th International Conference Learning Representations 2018 Conference Track Proceeding.
28. Rao ZMS, Malik N, 2012, Assessing iPSC Reprogramming Methods for their Suitability in Translational Medicine. J Cell Biochem, 113:3061–8. DOI: 10.1002/jcb.24183.
29. Kamath A, Ternes A, McGowan S, et al., 2017, Efficient Method to Create Integration-free, Virus-free, Myc and Lin28-free Human Induced Pluripotent Stem Cells from Adherent Cells. Future Sci OA, 3:FSO211. DOI: 10.4155/fsoa-2017-0028.
30. Malik N, Rao MS, 2013, A Review of the Methods for Human iPSC Derivation. Methods Mol Biol, 997:23–33.
31. Omole AE, Fakoya AO, 2018, Ten Years of Progress and Promise of Induced Pluripotent Stem Cells: Historical Origins, Characteristics, Mechanisms, Limitations, and Potential Applications. PeerJ, 6:e4370. DOI: 10.7717/peerj.4370.
32. Shi Y, Inoue H, Wu JC, et al., 2017, Induced Pluripotent Stem Cell Technology: A Decade of Progress. Nat Rev Drug Discov, 16:115–30. DOI: 10.1038/nrd.2016.245.
33. Yamanaka S, 2012, Induced Pluripotent Stem Cells: Past, Present, and Future. Cell Stem Cell, 10:678–84. DOI: 10.1016/j.stem.2012.05.005.
34. Takahashi K, Tanabe K, Ohnuki M, et al., 2007, Yamanaka, Induction of Pluripotent Stem Cells from Adult Human Fibroblasts by Defined Factors. Cell, 131:861–72. DOI: 10.1016/j.cell.2007.11.019.
35. Schlaeger TM, Daheron L, Brickler TR, et al., 2015, A Comparison of Non-integrating Reprogramming Methods. Nat Biotechnol, 33:58–63. DOI: 10.1038/nbt.3070.
36. Junying Y, Kejin H, Kim SO, et al., 2009, Human Induced Pluripotent Stem Cells Free of Vector and Transgene Sequences. Science, 324:797–801. DOI: 10.1126/science.1172482.
37. Shao L, Fujii H, Colmegna I, et al., 2009, Deficiency of the DNA Repair Enzyme ATM in Rheumatoid Arthritis. J Exp Med, 206:1435–49. DOI: 10.1084/jem.20082251.
38. Carey BW, Markoulaki A, Hanna J, et al., 2009, Reprogramming of Murine and Human Somatic Cells Using a Single Polycistronic Vector. Proc Natl Acad Sci USA, 106:157–62. DOI: 10.1073/pnas.0811426106.
39. McGrath PS, Diette N, Kogut I, et al., 2018, RNA-based Reprogramming of Human Primary Fibroblasts into Induced Pluripotent Stem Cells. J Vis Exp, 141:58687. DOI: 10.3791/58687.
40. Kogut I, McCarthy SM, Pavlova M, et al., 2018, High-efficiency RNA-based Reprogramming of Human Primary Fibroblasts. Nat Commun, 9:745. DOI: 10.1038/s41467-018-03190-3.
41. Qiao S, Deng Y, Li S, et al., 2019, Partially Reprogrammed Induced Pluripotent Stem Cells Using MicroRNA Cluster miR-302s in Guangxi Bama Minipig Fibroblasts. Cell Reprogram, 21:229–37. DOI: 10.1089/cell.2019.0035.
42. Baker M, 2009, MicroRNAs Boost Reprogramming, Boot Out cMyc. Nat Reports Stem Cells, 1:46. DOI: 10.1038/stemcells.2009.62.
43. Hirschi KK, Li S, Roy K, 2014, Induced Pluripotent Stem Cells for Regenerative Medicine. Annu Rev Biomed Eng, 16:277–94. DOI: 10.1146/annurev-bioeng-071813-105108.
44. Worringer KA, Rand TA, Hayashi Y, et al., 2014, The Let-7/LIN-41 Pathway Regulates Reprogramming to Human Induced Pluripotent Stem Cells by Controlling Expression of Prodifferentiation Genes. Cell Stem Cell, 14:40–52. DOI: 10.1016/j.stem.2013.11.001.
45. Kim D, Kim CH, J. Il Moon, et al., 2009, Generation of Human Induced Pluripotent Stem Cells by Direct Delivery of Reprogramming Proteins. Cell Stem Cell, 4:472–6. DOI: 10.1016/j.stem.2009.05.005.
46. Zhou H, Wu S, Joo JY, et al., 2009, Generation of Induced Pluripotent Stem Cells Using Recombinant Proteins. Cell Stem Cell, 4:381–4. DOI: 10.1016/j.stem.2009.04.005.
47. Seo BJ, Hong YJ, Do JT, 2017, Cellular Reprogramming Using Protein and Cell-Penetrating Peptides. Int J Mol Sci, 18:552. DOI: 10.3390/ijms18030552.
48. Lin T, Wu S, 2015, Reprogramming with Small Molecules Instead of Exogenous Transcription Factors. Stem Cells Int, 2015:794632. DOI: 10.1155/2015/794632.
49. Huangfu D, Maehr R, Guo W, et al., 2008, Induction of Pluripotent Stem Cells by Defined Factors is Greatly Improved by Small-molecule Compounds. Nat Biotechnol, 26:795–7. DOI: 10.1038/nbt1418.
50. Shi Y, Desponts C, Do JT, et al., 2008, Induction of Pluripotent Stem Cells from Mouse Embryonic Fibroblasts by Oct4 and Klf4 with Small-Molecule Compounds. Cell Stem Cell, 3:568–74. DOI: 10.1016/j.stem.2008.10.004.
51. Vijayavenkataraman S, Kannan S, Cao T, et al., 2019, 3D-Printed PCL/PPy Conductive Scaffolds as Three-Dimensional Porous Nerve Guide Conduits (NGCs) for Peripheral Nerve Injury Repair. Front Bioeng Biotechnol, 7:266. DOI: 10.3389/fbioe.2019.00266.
52. Hou P, Li Y, Zhang X, et al., 2013, Pluripotent Stem Cells Induced from Mouse Somatic Cells by Small molecule Compounds. Science, 341:651–4. DOI: 10.1126/science.1239278.
53. Zhang Y, Hu W, Ma K, et al., 2019, Reprogramming of Keratinocytes as Donor or Target Cells Holds Great Promise for Cell Therapy and Regenerative Medicine. Stem Cell Rev Reports, 15:680–9. DOI: 10.1007/s12015-019-09900-8.
54. Aasen T, Raya A, Barrero MJ, et al., 2008, Efficient and Rapid Generation of Induced Pluripotent Stem Cells from Human Keratinocytes. Nat Biotechnol, 26:1276–84. DOI: 10.1038/nbt.1503.
55. Sun N, Panetta NJ, Gupta DM, et al., 2009, Feeder-free Derivation of Induced Pluripotent Stem Cells from Adult Human Adipose Stem Cells. Proc Natl Acad Sci USA, 106:15720–5. DOI: 10.1073/pnas.0908450106.
56. Medvedev SP, Shevchenko AI, Zakian SM, 2010, Induced Pluripotent Stem Cells: Problems and Advantages when Applying them in Regenerative Medicine. Acta Nat, 2:18–27. DOI: 10.32607/20758251-2010-2-2-18-27.
57. Kim Y, Rim YA, Yi H, et al., 2016, The Generation of Human Induced Pluripotent Stem Cells from Blood Cells: An Efficient Protocol Using Serial Plating of Reprogrammed Cells by Centrifugation. Stem Cells Int, 2016:1329459. DOI: 410.1155/2016/1329459.
58. Okumura T, Horie Y, Lai CY, et al., 2019, Robust and Highly Efficient hiPSC Generation from Patient Non-mobilized Peripheral Blood-derived CD34+ Cells Using the Autoerasable Sendai Virus Vector. Stem Cell Res Ther, 10:185. DOI: 10.1186/s13287-019-1273-2.
59. Corbett JL, Duncan SA, 2019, iPSC-Derived Hepatocytes as a Platform for Disease Modeling and Drug Discovery. Front Med, 6:265. DOI: 10.3389/fmed.2019.00265.
60. Kwong G, Marquez HA, Yang C, et al., 2019, Generation of a Purified iPSC-Derived Smooth Muscle-like Population for Cell Sheet Engineering. Stem Cell Reports, 13:499–514. DOI: 10.1016/j.stemcr.2019.07.014.
61. Abdul Manaph NP, Sivanathan KN, Nitschke J, et al., 2019, An Overview on Small Molecule-induced Differentiation of Mesenchymal Stem Cells Into Beta Cells for Diabetic Therapy. Stem Cell Res Ther, 10:2194. DOI: 10.1186/s13287-019-1396-5.
62. Tabar V, Studer L, 2014, Pluripotent Stem Cells in Regenerative Medicine: Challenges and Recent Progress. Nat Rev Genet, 15:82–92. DOI: 10.1038/nrg3563.
63. Kang H, Shih YR, Nakasaki M, et al., Small Molecule Driven Direct Conversion of Human Pluripotent Stem Cells into Functional Osteoblasts. Sci Adv, 2:e1600691. DOI: 10.1126/sciadv.1600691.
64. Ong CS, Yesantharao P, Huang CY, et al., 2018, 3D Bioprinting Using Stem Cells. Pediatr Res, 83:223–31.
65. Ozbolat IT, Hospodiuk M, 2016, Current Advances and Future Perspectives in Extrusion-based Bioprinting. Biomaterials, 76:321–43. DOI: 10.1016/j.biomaterials.2015.10.076.
66. Reid JA, Mollica PA, Johnson GD, et al., 2016, Accessible Bioprinting: Adaptation of a Low-cost 3D-Printer for Precise Cell Placement and Stem Cell Differentiation. Biofabrication, 8:025017. DOI: 10.1088/1758-5090/8/2/025017.
67. Gu Q, Tomaskovic-Crook E, Wallace GG, et al., 2017, 3D Bioprinting Human Induced Pluripotent Stem Cell Constructs for In Situ Cell Proliferation and Successive Multilineage Differentiation. Adv Healthc Mater, 6:1700175. DOI: 10.1002/adhm.201700175.
68. Colosi S, Soloperto B, Benedetti T, et al., 2019, 3D Bioprinted Human Cortical Neural Constructs Derived from Induced Pluripotent Stem Cells. J Clin Med, 8:1595. DOI: 10.3390/jcm8101595.
69. Nguyen D, Hägg DA, Forsman A, et al., 2017, Cartilage Tissue Engineering by the 3D Bioprinting of iPS Cells in a Nanocellulose/Alginate Bioink. Sci Rep, 7:6684. DOI: 10.1038/s41598-017-00690-y.
70. Skylar-Scott MA, Uzel SG, Nam LL, et al., 2019, Biomanufacturing of Organ-Specific Tissues with High Cellular Density and Embedded Vascular Channels. Sci Adv, 5:eaaw2459. DOI: 10.1126/sciadv.aaw2459.
71. Zhang C, Yang F, Cornelia R, et al., 2011, Hypoxia-inducible Factor-1 is a Positive Regulator of Sox9 Activity in Femoral Head Osteonecrosis. Bone, 48:507–13. DOI: 10.1016/j.bone.2010.10.006.
72. Sun AX, Lin H, Beck AM, et al., 2015, Projection Stereolithographic Fabrication of Human Adipose Stem Cell-Incorporated Biodegradable Scaffolds for Cartilage Tissue Engineering. Front Bioeng Biotechnol, 3:115. DOI: 10.3389/fbioe.2015.00115.
73. Ma X, Qu X, Zhu W, et al., 2016, Deterministically Patterned Biomimetic Human iPSC-Derived Hepatic Model Via Rapid 3D Bioprinting. Proc Natl Acad Sci, 113:2206–11. DOI: 10.1073/pnas.1524510113.
74. Fairbanks BD, Schwartz MP, Bowman CN, et al., 2009, Photoinitiated Polymerization of PEG-diacrylate with Lithium Phenyl-2,4,6-Trimethylbenzoylphosphinate: Polymerization Rate and Cytocompatibility. Biomaterials, 30:6702–7. DOI: 10.1016/j.biomaterials.2009.08.055.
75. Ng WL, Lee JM, Zhou M, et al., 2020, Vat Polymerization-Based Bioprinting Process, Materials, Applications and Regulatory Challenges. Biofabrication, 12:022001. DOI: 410.1088/1758-5090/ab6034.
76. Fattah AR, Meleca E, Mishriki S, et al., 2016, In Situ 3D Label-Free Contactless Bioprinting of Cells through Diamagnetophoresis. ACS Biomater Sci Eng, 2:2133–8. DOI: 10.1021/acsbiomaterials.6b00614.
77. Gudapati H, Dey M, Ozbolat I, 2016, A Comprehensive Review on Droplet-based Bioprinting: Past, Present and Future. Biomaterials, 102:20–42. DOI: 10.1016/j.biomaterials.2016.06.012.
78. Guillotin B, Souquet A, Catros S, et al., 2010, Laser Assisted Bioprinting of Engineered Tissue with High Cell Density and Microscale Organization. Biomaterials, 31:7250–6. DOI: 10.1016/j.biomaterials.2010.05.055.
79. Li Y, Jiang X, Li L, et al., 2018, 3D Printing Human Induced Pluripotent Stem Cells with Novel Hydroxypropyl Chitin Bioink: Scalable Expansion and Uniform Aggregation. Biofabrication, 10:044101. DOI: 10.1088/1758-5090/aacfc3.
80. Ng WL, Chua CK, Shen YF, 2019, Print Me An Organ! Why We Are Not There Yet. Prog Polym Sci, 97:101145. DOI: 10.1016/j.progpolymsci.2019.101145.
81. Romanazzo S, Nemec S, Roohani I, 2019, iPSC Bioprinting: Where are we at? Materials (Basel), 12:2453. DOI: 10.3390/ma12152453.
82. McCauley HA, Wells JM, 2017, Pluripotent Stem Cellderived Organoids: Using Principles of Developmental Biology to Grow Human Tissues in a Dish. Development, 144:958–62. DOI: 10.1242/dev.140731.
83. Liu C, Oikonomopoulos A, Sayed N, et al., 2018, Modeling Human Diseases with Induced Pluripotent Stem Cells: From 2D to 3D and Beyond. Development, 145:dev156166. DOI: 10.1242/dev.156166.
84. Zhang B, Gao L, Ma L, et al., 2017, 3D Bioprinting: A Novel Avenue for Manufacturing Tissues and Organs. Engineering, 5:777–94.
85. Varkey M, Visscher DO, van Zuijlen PP, et al., 2019, Skin Bioprinting: The Future of Burn Wound Reconstruction? Burn Trauma, 7:8171. DOI: 10.1186/s41038-019-0142-7.
86. Kamel RA, Ong JF, Eriksson E, et al., 2013, Tissue Engineering of Skin. J Am Coll Surg, 217:533–55.
87. Yang B, Lui C, Yeung E, et al., 2019, A Net Mold-Based Method of Biomaterial-Free Three-Dimensional Cardiac Tissue Creation. Tissue Eng Part C Methods, 25:243–52. DOI: 10.1089/ten.tec.2019.0003.
88. Vijayavenkataraman S, Kannan S, Cao T, et al., 2019, 3D-Printed PCL/PPy Conductive Scaffolds as Three-Dimensional Porous Nerve Guide Conduits (NGCs) for Peripheral Nerve Injury Repair. Front Bioeng Biotechnol, 7:266. DOI: 10.3389/fbioe.2019.00266.
89. Chen YM, Chen LH, Li MP, et al., 2017, Xeno-free Culture of Human Pluripotent Stem Cells on Oligopeptide-Grafted Hydrogels with Various Molecular Designs. Sci Rep, 7:45146. DOI: 10.1038/srep45146.
90. Wiley LA, Anfinson KR, Cranston CM, et al., 2017, Generation of Xeno-Free, cGMP-Compliant Patient-Specific iPSCs from Skin Biopsy. Curr Protoc Stem Cell Biol, 42:4A.12.1–4A.12.4. Doi: 10.1002/cpsc.30.
91. Pruksananonda K, Rungsiwiwut R, 2016, Moving toward xeno-free culture of human pluripotent stem cells. In: Pluripotent Stem Cells: From the Bench to the Clinic. BoD-Books on Demand, Norderstedt, Germany. DOI: 10.5772/62770.
92. Boreström C, Simonsson S, Enochson L, et al., 2014, Footprint-Free Human Induced Pluripotent Stem Cells From Articular Cartilage With Redifferentiation Capacity: A First Step Toward a Clinical-Grade Cell Source. Stem Cells Transl Med, 3:433–47. DOI: 10.5966/sctm.2013-0138.
93. Attwood S, Edel M, 2019, iPS-Cell Technology and the Problem of Genetic Instability can it ever be Safe for Clinical Use? J Clin Med, 8:288. DOI: 10.3390/jcm8030288.
94. Matz P, Wruck W, Fauler B, et al., 2017, Footprint-free Human Fetal Foreskin Derived iPSCs: A Tool for Modeling Hepatogenesis Associated Gene Regulatory Networks. Sci Rep, 7:310. DOI: 10.1038/s41598-017-06546-9.
95. Atkinson MA, Eisenbarth GS, Michels AW, 2014, Type 1 Diabetes. Lancet, 383:69–82.
96. Katsarou A, Gudbjörnsdottir S, Rawshani A, et al., 2017, Type 1 Diabetes Mellitus. Nat Rev Dis Prim, 3:17016.
97. Kim J, Shim IK, Hwang DG, et al., 2019, 3D Cell Printing of Islet-laden Pancreatic Tissue-derived Extracellular Matrix Bioink Constructs for Enhancing Pancreatic Functions. J Mater Chem B, 7:1773–81. DOI: 10.1039/c8tb02787k.
98. Taalesen H, Ketner MA, 2018, Milestone in Development of 3D Bioprinting of Biomimetic Pancreas to Treat Diabetes. University of Oslo, Hybrid Technology Hub, Oslo. Available from: https://www.med.uio.no/hth/english/news-and-events/news/3dbioprinting.html. [Last accessed on 2020 Jun 20].
99. Davies S, 2016, Successfully 3D Print Functioning Pancreas Model. Mag Des Innovations. Celprogen Inc., Torrance.
100. Ebrahimi M, Asbagh FA, 2011, Pathogenesis and Causes of Premature Ovarian Failure: An Update. Int J Fertil Steril, 5:54–65.
101. Laronda MM, Rutz AL, Xiao S, et al., 2017, A Bioprosthetic Ovary Created Using 3D Printed Microporous Scaffolds Restores Ovarian Function in Sterilized Mice. Nat Commun, 8:15261. DOI: 10.1038/ncomms15261.
102. Irvine SA, Agrawal A, Lee BH, et al., 2015, Printing cell laden gelatin constructs by free-form fabrication and enzymatic protein crosslinking. Biomed Microdevices, 17:16. DOI: 10.1007/s10544-014-9915-8.
103. Bulanova EA, Koudan EV, Degosserie J, et al., 2017, Bioprinting of a Functional Vascularized Mouse Thyroid Gland Construct. Biofabrication, 9:034105. DOI: 10.1088/1758-5090/aa7fdd.
104. International Space Station Program Science Forum, 2018, International Space Station Benefits for Humanity. 3rd ed. NASA. Available from: https://www.nasa.gov/sites/default/files/atoms/files/benefits-for-humanity_third.pdf. [Last accessed on 2020 Jun 20].
105. Hamazaki T, El Rouby N, Fredette NC, et al., 2017, Concise Review: Induced Pluripotent Stem Cell Research in the Era of Precision Medicine. Stem Cells, 35:545–50. DOI: 10.1002/stem.2570.
106. Walker C, Mojares E, Del Río Hernández A, 2018, Role of Extracellular Matrix in Development and Cancer Progression. Int J Mol Sci, 19:3028. DOI: 10.3390/ijms19103028.
107. Ma X, Liu J, Zhu W, et al., 2018, 3D Bioprinting of Functional Tissue Models for Personalized Drug Screening and In Vitro Disease Modeling. Adv Drug Deliv Rev, 132:235–51. DOI: 10.1016/j.addr.2018.06.011.
108. Antill-O’Brien N, Bourke J, O’Connell CD, 2019, Layer-by-layer: The case for 3D bioprinting neurons to create patient-specific epilepsy models. Materials (Basel), 12:3218. DOI: 10.3390/ma12193218.
109. Alonzo M, AnilKumar S, Roman B, et al., 2019, 3D Bioprinting of Cardiac Tissue and Cardiac Stem Cell Therapy. Transl Res, 211:64–83. DOI: 10.1016/j.trsl.2019.04.004.
110. Zimmermann WH, Cesnjevar R, 2009, Cardiac Tissue Engineering: Implications for Pediatric Heart Surgery. Pediatr Cardiol, 30:716–23. DOI: 10.1007/s00246-009-9405-6.
111. Tzatzalos E, Abilez OJ, Shukla P, et al., 2016, Engineered Heart Tissues and Induced Pluripotent Stem Cells: Macro and Microstructures for Disease Modeling, Drug Screening, and Translational Studies. Adv Drug Deliv Rev, 96:234–44. DOI: 10.1016/j.addr.2015.09.010.
112. Yoshida Y, Yamanaka S, 2017, Induced Pluripotent Stem Cells 10 Years Later. Circ Res, 120:1958–68. DOI: 10.1161/circresaha.117.311080.
113. Hinson JT, Chopra A, Nafissi N, et al., 2015, Titin Mutations in iPS Cells Define Sarcomere Insufficiency as a Cause of Dilated Cardiomyopathy. Science, 349:982–6. DOI: 10.1126/science.aaa5458.
114. Tiburcy M, Hudson JE, Balfanz P, et al., 2017, Defined Engineered Human Myocardium with Advanced Maturation for Applications in Heart Failure Modeling and Repair. Circulation, 135:1832–47.
115. Takebe T, Zhang B, Radisic M, 2017, Synergistic Engineering: Organoids Meet Organs-on-a-Chip. Cell Stem Cell, 21:297–300. DOI: 10.1016/j.stem.2017.08.016.
116. Bhatia SN, Ingber DE, 2014, Microfluidic Organs-on-chips. Nat Biotechnol, 32:760–72. DOI: 10.1038/nbt.2989.
117. Huh D, Matthews BD, Mammoto A, et al., 2010, Ingber, Reconstituting Organ-level Lung Functions on a Chip. Science, 328:1662–8. DOI: 10.1126/science.1188302.
118. Low LA, Tagle DA, 2017, Tissue Chips-innovative Tools for Drug Development and Disease Modeling. Lab Chip, 17:3026–36. DOI: 10.1039/c7lc00462a.
119. Eiraku M, Watanabe K, Matsuo-Takasaki M, et al., 2008, Self-Organized Formation of Polarized Cortical Tissues from ESCs and its Active Manipulation by Extrinsic Signals. Cell Stem Cell, 3:519–32. DOI: 10.1016/j.stem.2008.09.002.
120. Montine TJ, Phelps CH, Beach TG, et al., 2012, National Institute on Aging-Alzheimer’s Association Guidelines for the Neuropathologic Assessment of Alzheimer’s Disease: A Practical Approach. Acta Neuropathol, 123:1–11. DOI: 10.1007/s00401-011-0910-3.
121. van Giau V, Lee H, Shim KH, et al., 2018, Genome-editing Applications of CRISPR Cas9 to Promote In Vitro Studies of Alzheimer’s Disease. Clin Interv Aging, 13:221–33.
122. De Strooper B, Karran E, 2016, The Cellular Phase of Alzheimer’s Disease. Cell, 164:603–15. DOI: 10.1016/j.cell.2015.12.056.
123. Vijayavenkataraman S, Thaharah S, Zhang S, et al., 2019, 3D-Printed PCL/rGO Conductive Scaffolds for Peripheral Nerve Injury Repair. Artif Organs, 43:515–23. DOI: 10.1111/aor.13360.
124. Chambers SM, Fasano CA, Papapetrou EP, et al., 2009, Highly Efficient Neural Conversion of Human ES and iPS Cells by Dual Inhibition of SMAD Signaling. Nat Biotechnol, 27:275–80. DOI: 10.1038/nbt.1529.
125. Ortiz-Virumbrales M, Moreno CL, Kruglikov I, et al., 2017, CRISPR/Cas9-Correctable Mutation-related Molecular and Physiological Phenotypes in iPSC-Derived Alzheimer’s PSEN2N141I Neurons. Acta Neuropathol Commun, 5:77. DOI: 10.1186/s40478-017-0475-z.
126. Drummond E, Wisniewski T, 2017, Alzheimer’s Disease: Experimental Models and Reality. Acta Neuropathol, 133:155–75. DOI: 10.1007/s00401-016-1662-x.
127. Li H, Jiang H, Zhang B, et al., 2018, Modeling Parkinson’s Disease Using Patient-specific Induced Pluripotent Stem Cells. J Parkinsons Dis, 8:479–93.
128. Torrent R, De Angelis Rigotti F, Dell’Era P, et al., 2015, Using iPS Cells Toward the Understanding of Parkinson’s Disease. J Clin Med, 4:548–66. DOI: 10.3390/jcm4040548.
129. Zeng XS, Geng WS, Jia JJ, 2018, Neurotoxin-Induced Animal Models of Parkinson Disease: Pathogenic Mechanism and Assessment. ASN Neuro, 10:1759091418777438. DOI: 10.1177/1759091418777438.
130. Bordoni M, Rey F, Fantini V, et al., 2018, From Neuronal Differentiation of iPSCs to 3D Neuro-organoids: Modelling and Therapy of Neurodegenerative Diseases. Int J Mol Sci, 19:3972. DOI: 10.3390/ijms19123972.
131. Thomas M, Willerth SM, 2017, 3-D Bioprinting of Neural Tissue for Applications in Cell Therapy and Drug Screening. Front Bioeng Biotechnol, 5:69. DOI: 10.3389/fbioe.2017.00069.
132. Osborn TM, Beagan J, Isacson O, 2018, Increased Motor Neuron Resilience by Small Molecule Compounds that Regulate IGF-II Expression. Neurobiol Dis, 110:218–30. DOI: 10.1016/j.nbd.2017.11.002.
133. Osaki T, Uzel SG, Kamm RD, 2018, Microphysiological 3D Model of Amyotrophic Lateral Sclerosis (ALS) from Human iPS-derived Muscle Cells and Optogenetic Motor Neurons. Sci Adv, 4:eaat5847. DOI: 10.1126/sciadv.aat5847.
134. Câmara DA, Mambelli LI, Porcacchia AS, et al., 2016, Advances and Challenges on Cancer Cells Reprogramming Using Induced Pluripotent Stem Cells Technologies. J Cancer, 7:2296–303. DOI: 10.7150/jca.16629.
135. Papapetrou EP, 2016, Patient-derived Induced Pluripotent Stem Cells in Cancer Research and Precision Oncology. Nat Med, 22:1392–401. DOI: 10.1038/nm.4238.
136. Kotini AG, Chang CJ, Chow A, et al., 2017, Stage-Specific Human Induced Pluripotent Stem Cells Map the Progression of Myeloid Transformation to Transplantable Leukemia. Cell Stem Cell, 20:315–28. DOI: 10.1016/j.stem.2017.01.009.
137. Hu K, Yu J, Suknuntha K, et al., 2011, Efficient Generation of Transgene-free induced Pluripotent Stem Cells from Normal and Neoplastic Bone Marrow and Cord Blood Mononuclear Cells. Blood, 117: e109–19. DOI: 10.1182/blood-2010-07-298331.
138. Gandre-Babbe S, Paluru P, Aribeana C, et al., 2013, Patient derived Induced Pluripotent Stem Cells Recapitulate Hematopoietic Abnormalities of Juvenile Myelomonocytic Leukemia. Blood, 121:4925–9. DOI: 10.1182/blood-2013-01-478412.
139. Kotini AG, Chang CJ, Boussaad I, et al., 2015, Functional Analysis of a Chromosomal Deletion Associated with Myelodysplastic Syndromes using Isogenic Human Induced Pluripotent Stem Cells. Nat Biotechnol, 33:646–55. DOI: 10.1038/nbt.3178.
140. Fang Y, Eglen RM, 2017, Three-Dimensional Cell Cultures in Drug Discovery and Development. SLAS Discov, 22:456–72. DOI: 10.1177/1087057117696795.
141. Senthebane DA, Rowe A, Thomford NE, et al., 2017, The Role of Tumor Microenvironment in Chemoresistance: To Survive, Keep your Enemies Closer. Int J Mol Sci, 18:1586. DOI: 10.3390/ijms18071586.
142. Abbott A, 2019, The Lowdown on Animal Testing for Cosmetics. Nature, 2:496.
143. Skardal A, Mack D, Kapetanovic E, et al., 2012, Bioprinted Amniotic Fluid-Derived Stem Cells Accelerate Healing of Large Skin Wounds. Stem Cells Transl Med, 1:792–802. DOI: 10.5966/sctm.2012-0088/.
144. Teoh JH, Thamizhchelvan AM, Davoodi P, et al., 2019, Investigation of the Application of a Taylor-Couette Bioreactor in the Post-processing of Bioprinted Human Dermal Tissue. Biochem Eng J, 151:107317. DOI: 10.1016/j.bej.2019.107317.
145. Kim BS, Lee JS, Gao G, et al., 2017, Direct 3D Cell printing of Human Skin with Functional Transwell System. Biofabrication, 9:025034. DOI: 10.1088/1758-5090/aa71c8.
146. Gentile P, McColgan-Bannon K, Gianone NC, et al., 2017, Biosynthetic PCL-Graft-collagen Bulk Material for Tissue Engineering Applications. Materials (Basel), 10:693. DOI: 10.3390/ma10070693.
147. Ashammakhi N, Ahadian S, Xu C, et al., 2019, Bioinks and Bioprinting Technologies to Make Heterogeneous and Biomimetic tissue Constructs. Mater Today Bio, 1:100008. DOI: 10.1016/j.mtbio.2019.100008.
148. Mosadegh B, Xiong G, Dunham S, et al., 2015, Current Progress in 3D Printing for Cardiovascular Tissue Engineering. Biomed Mater (Bristol), 10:034002. DOI: 10.1088/1748-6041/10/3/034002.
149. Mandrycky C, Wang Z, Kim K, et al., 2016, 3D Bioprinting for Engineering Complex Tissues. Biotechnol Adv, 34:422–34. DOI: 10.1016/j.biotechadv.2015.12.011.
150. Zhang XF, Huang Y, Gao G, et al., 2017, Current Progress in Bioprinting. In: Tripathi A, Melo JS, editor. Advances in Biomaterials for Biomedical Applications. Springer, Singapore, pp. 227–59. DOI: 10.1016/j.mtbio.2019.100008.
151. Skardal A, Atala A, 2015, Biomaterials for Integration with 3-D Bioprinting. Annals of Biomedical Engineering, 43:730–46. DOI: 10.1007/s10439-014-1207-1.
152. Billiet T, Vandenhaute M, Schelfhout J, et al., 2012, A Review of Trends and Limitations in Hydrogel-rapid Prototyping for Tissue Engineering. Biomaterials, 33:6020-41. DOI: 10.1016/j.biomaterials.2012.04.050.
153. Zhao T, Zhang ZN, Rong Z, et al., 2011, Immunogenicity of Induced Pluripotent Stem Cells. Nature, 474:212–6. DOI: 10.1038/nature10135.
154. Vijayavenkataraman S, Yan WC, Lu WF, et al., 2018, 3D Bioprinting of Tissues and Organs for Regenerative Medicine. Adv Drug Deliv Rev, 132:296–332. DOI: 10.1016/j.addr.2018.07.004.
155. Zhang S, Vijayavenkataraman S, Lu WF, et al., 2019, A Review on the Use of Computational Methods to Characterize, Design, and Optimize Tissue Engineering Scaffolds, with a Potential in 3D Printing Fabrication. J Biomed Mater Res Part B Appl Biomater, 107:1329–51. DOI: 10.1002/jbm.b.34226.