3D Freeform Printing of Nanocomposite Hydrogels through in situ Precipitation in Reactive Viscous Fluid
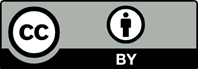
Composite hydrogels have gained great attention as three-dimensional (3D) printing biomaterials because of their enhanced intrinsic mechanical strength and bioactivity compared to pure hydrogels. In most conventional printing methods for composite hydrogels, particles are preloaded in ink before printing, which often reduces the printability of composite ink with little mechanical improvement due to poor particle-hydrogel interaction of physical mixing. In contrast, the in situ incorporation of nanoparticles into a hydrogel during 3D printing achieves uniform distribution of particles with remarkable mechanical reinforcement, while precursors dissolved in inks do not influence the printing process. Herein, we introduced a “printing in liquid” technique coupled with a hybridization process, which allows 3D freeform printing of nanoparticle-reinforced composite hydrogels. A viscoplastic matrix for this printing system provides not only support for printed hydrogel filaments but also chemical reactants to induce various reactions in printed objects for in situ modification. Nanocomposite hydrogel scaffolds were successfully fabricated through this 3D freeform printing of hyaluronic acid (HAc)-alginate (Alg) hydrogel inks through a two-step crosslinking strategy. The first ionic crosslinking of Alg provided structural stability during printing, while the secondary crosslinking of photo-curable HAc improved the mechanical and physiological stability of the nanocomposite hydrogels. For in situ precipitation during 3D printing, phosphate ions were dissolved in the hydrogel ink and calcium ions were added to the viscoplastic matrix. The composite hydrogels demonstrated a significant improvement in mechanical strength, biostability, as well as biological performance compared to pure HAc. Moreover, the multi-material printing of composites with different calcium phosphate contents was achieved by adjusting the ionic concentration of inks. Our method greatly accelerates the 3D printing of various functional or hybridized materials with complex geometries through the design and modification of printing materials coupled with in situ post-printing functionalization and hybridization in reactive viscoplastic matrices.
1. Liu W, Zhang YS, Heinrich MA, et al., 2017, Rapid Continuous Multimaterial Extrusion Bioprinting. Adv Mater, 29:1604630.
2. Kowsari K, Akbari S, Wang D, et al., 2018, High-efficiency High-resolution Multimaterial Fabrication for Digital Light Processing-based Three-dimensional Printing. 3D Print Addit Manuf, 5:185–93. DOI: 10.1089/3dp.2018.0004.
3. Lopes LR, Silva AF, Carneiro OS, 2018, Multi-material 3D Printing: The Relevance of Materials Affinity on the Boundary Interface Performance. Addit Manuf, 23:45–52. DOI: 10.1016/j.addma.2018.06.027.
4. Jang TS, Jung HD, Pan HM, et al., 2018, 3D Printing of Hydrogel Composite Systems: Recent Advances in Technology for Tissue Engineering. Int J Bioprinting, 4:126.
5. Jeong SH, Koh YH, Kim SW, et al., 2016, Strong and Biostable Hyaluronic Acid-calcium Phosphate Nanocomposite Hydrogel via In Situ Precipitation Process. Biomacromolecules, 17:841–51. DOI: 10.1021/acs.biomac.5b01557.
6. Wust S, Godla ME, Muller R, et al., 2014, Tunable Hydrogel Composite with Two-step Processing in Combination with Innovative Hardware Upgrade for Cell-based Three-dimensional Bioprinting. Acta Biomater, 10:630–40. DOI:10.1016/j.actbio.2013.10.016.
7. Thoniyot P, Tan MJ, Karim AA, et al., 2015, Nanoparticlehydrogel Composites: Concept, Design, and Applications of These Promising, Multi-functional Materials. Adv Sci (Weinh), 2:1400010. DOI: 10.1002/advs.201400010.
8. Gaharwar AK, Schexnailder PJ, Dundigalla A, et al., 2011, Highly Extensible Bio-nanocomposite Fibers. Macromol Rapid Commun, 32:50–7. DOI: 10.1002/marc.201000556.
9. Leach JB, Bivens KA, Patrick CW, et al., 2003, Photocrosslinked Hyaluronic Acid Hydrogels: Natural,
Biodegradable Tissue Engineering Scaffolds. Biotechnol Bioeng, 82:578–89. DOI: 10.1002/bit.10605.
10. Xu X, Jha AK, Harrington DA, et al., 2012, Hyaluronic Acid-based Hydrogels: From a Natural Polysaccharide to Complex Networks. Soft Matter, 8:3280–94. DOI: 10.1039/c2sm06463d.
11. Kisiel M, Martino MM, Ventura M, et al., 2013, Improving the Osteogenic Potential of BMP-2 with Hyaluronic Acid Hydrogel Modified with Integrin-specific Fibronectin Fragment. Biomaterials, 34:704–12. DOI: 10.1016/j.biomaterials.2012.10.015.
12. Li QH, Li M, Zhu PZ, et al., 2012, In Vitro Synthesis of Bioactive Hydroxyapatite Using Sodium Hyaluronate as a Template. J Mater Chem, 22:20257–65. DOI: 10.1039/c2jm33624c.
13. Egorov AA, Fedotov AY, Mironov AV, et al., 2016, 3D Printing of Mineral-polymer Bone Substitutes Based on Sodium Alginate and Calcium Phosphate. Beilstein J Nanotechnol, 7:1794–9. DOI: 10.3762/bjnano.7.172.
14. Lee H, Kim Y, Kim S, et al., 2014, Mineralized Biomimetic Collagen/Alginate/Silica Composite Scaffolds Fabricated by a Low-temperature Bio-plotting Process for Hard Tissue Regeneration: Fabrication, Characterisation and In Vitro Cellular Activities. J Mater Chem B, 2:5785. DOI: 10.1039/c4tb00931b.
15. Bhattacharjee T, Zehnder SM, Rowe KG, et al., 2015, Writing in the Granular Gel Medium. Sci Adv, 1:e1500655.DOI: 10.1126/sciadv.1500655.
16. Highley CB, Rodell CB, Burdick JA, 2015, Direct 3D Printing of Shear-thinning Hydrogels into Self-healing Hydrogels. Adv Mater, 27:5075–9. DOI: 10.1002/adma.201501234.
17. Hinton TJ, Jallerat Q, Palchesko RN, et al., 2015, Three-dimensional Printing of Complex Biological Structures by Freeform Reversible Embedding of Suspended Hydrogels. Sci Adv, 1:e1500758. DOI: 10.1002/adma.201501234.
18. Hinton TJ, Hudson A, Pusch K, et al., 2016, 3D Printing PDMS Elastomer in a Hydrophilic Support Bath via Freeform Reversible Embedding. ACS Biomater Sci Eng, 2:1781–6. DOI: 10.1021/acsbiomaterials.6b00170.
19. Rodriguez MJ, Dixon TA, Cohen E, et al., 2018, 3D Freeform Printing of Silk Fibroin. Acta Biomater, 71:379–87. DOI: 10.1016/j.actbio.2018.02.035.
20. Grosskopf AK, Truby RL, Kim H, et al., 2018, Viscoplastic Matrix Materials for Embedded 3D Printing. ACS Appl Mater Interfaces, 10:23353–61. DOI: 10.1021/acsami.7b19818.
21. Pan HM, S, Jang TS, et al., 2019, Plant Seed inspired Cell Protection, Dormancy, and Growth for Largescale Biofabrication. Biofabrication, 11:025008. DOI: 10.1088/1758-5090/ab03ed.
22. Furuichi K, Oaki Y, Imai H, 2006, Preparation of Nanotextured and Nanofibrous Hydroxyapatite Through Dicalcium Phosphate with Gelatin. Chem Mater, 18:229–34. DOI: 10.1021/cm052213z.
23. Thanh NT, Maclean N, Mahiddine S, 2014, Mechanisms of Nucleation and Growth of Nanoparticles in Solution. Chem Rev, 114:7610–30. DOI: 10.1021/cr400544s.
24. Bastami A, Allahgholi M, Pourafshary P, 2014, Experimental and Modelling Study of the Solubility of CO2 in Various CaCl2 Solutions at Different Temperatures and Pressures. Pet Sci, 11:569–77. DOI: 10.1007/s12182-014-0373-1.
25. Hosoda N, Kato T, 2001, Thin-film Formation of Calcium Carbonate Crystals: Effects of Functional Groups of Matrix Polymers. Chem Mater, 13:688–93. DOI: 10.1021/cm000817r.
26. Gallagher PK, Johnson DW, 1973, The Effects of Sample Size and Heating Rate on the Kinetics of the Thermal Decomposition of CaCO3. Thermochim Acta, 6:67–83. DOI: 10.1016/0040-6031(73)80007-3.
27. Li ZY, Su YL, Xie BQ, et al., 2013, A Tough Hydrogelhydroxyapatite Bone-like Composite Fabricated In Situ by the Electrophoresis Approach. J Mater Chem B, 1:1755–64. DOI: 10.1039/c3tb00246b.
28. Sarvestani AS, He XZ, Jabbari E, 2008, The role of filler matrix interaction on viscoelastic response of biomimetic nanocomposite hydrogels. J Nanomater, 2008:9. DOI:10.1155/2008/126803.
29. Song X, Zhu C, Fan D, et al., 2017, A Novel Human-like Collagen Hydrogel Scaffold with Porous Structure and Sponge-like Properties. Polymers, 9:638. DOI: 10.3390/polym9120638.
30. Sarvestani AS, Jabbari E, 2008, A Model for the Viscoelastic Behavior of Nanofilled Hydrogel Composites Under Oscillatory Shear Loading. Polym Compos, 29:326–36. DOI: 10.1002/pc.20416.
31. Desimone MF, Helary C, Quignard S, et al., 2011, In Vitro Studies and Preliminary In Vivo Evaluation of Silicified Concentrated Collagen Hydrogels. ACS Appl Mater Interfaces, 3:3831–8. DOI: 10.1021/am2009844.
32. van Hoogmoed CG, Busscher HJ, de Vos P, 2003, Fourier Transform Infrared Spectroscopy Studies of Alginate-PLL Capsules with Varying Compositions. J Biomed Mater Res Part A, 67A:172–8. DOI: 10.1002/jbm.a.10086.
33. Ibrahim S, Kothapalli CR, Kang QK, et al., 2011, Characterization of Glycidyl Methacrylate-crosslinked Hyaluronan Hydrogel Scaffolds Incorporating Elastogenic Hyaluronan Oligomers. Acta Biomater, 7:653–65. DOI: 10.1016/j.actbio.2010.08.006.
34. Berzina-Cimdina L, Borodajenko N, 2012, Research of Calcium Phosphates Using Fourier Transform Infrared Spectroscopy. InTech, Rijeka. DOI: 10.5772/36942.
35. Dotzauer DM, Dai J, Sun L, et al., 2006, Catalytic Membranes Prepared Using Layer-by-layer Adsorption of Polyelectrolyte/Metal Nanoparticle Films in Porous Supports. Nano Lett, 6:2268–72. DOI: 10.1021/nl061700q.
36. Ahmed SR, Kim J, Tran VT, et al., 2017, In Situ Self-assembly of Gold Nanoparticles on Hydrophilic and Hydrophobic Substrates for Influenza Virus-sensing Platform. Sci Rep, 7:44495. Available from: https://www.nature.com/articles/srep44495#supplementary-information. DOI: 10.1038/srep44495.
37. Jakus AE, Taylor SL, Geisendo rfer NR, et al., 2015, Metallic Architectures from 3D-printed Powder-based Liquid Inks. Adv Funct Mater, 25:6985–95. DOI: 10.1002/adfm.201503921.
38. Murphy WL, Mooney DJ, 2002, Bioinspired Growth of Crystalline Carbonate Apatite on Biodegradable Polymer Substrata. J Am Chem Soc, 124:1910−7. DOI: 10.1021/ja012433n.
39. Discher DE, Janmey P, Wang YL, 2005, Tissue Cells Feel and Respond to the Stiffness of Their Substrate. Science, 310:1139−43. DOI: 10.1126/science.1116995.
40. Tamimi F, Sheikh Z, Barralet J, 2012, Dicalcium Phosphate Cements: Brushite and Monetite. Acta Biomater, 8:474–87. DOI: 10.1016/j.actbio.2011.08.005.
41. Han WT, Jang T, Chen S, et al., 2020, Improved Cell Viability for Large-scale Biofabrication with Photo-crosslinkable Hydrogel Systems Through a Dual-photoinitiator Approach. Biomater Sci, 8:450–61. DOI: 10.1039/c9bm01347d.
42. Huang W, Yang S, Shao J, et al., 2007, Signaling and Transcriptional Regulation in Osteoblast Commitment and Differentiation. Front Biosci J Virtual Lib, 12:3068–92. Doi: 10.2741/2296.
43. Huebsch N, Arany PR, Mao AS, et al., 2010, Harnessing Traction mediated Manipulation of the Cell/Matrix Interface to Control Stem-cell fate. Nat Mater, 9:518–26. DOI: 10.1038/nmat2732.
44. Bai X, Gao M, Syed S, et al., 2018, Bioactive Hydrogels for Bone Regeneration. Bioact Mater, 3:401–17.
45. Gaharwar AK, Mihaila SM, Swami A, et al., 2013, Bioactive Silicate Nanoplatelets for Osteogenic Differentiation of Human Mesenchymal Stem Cells. Adv Mater, 25:3329–36. DOI: 10.1002/adma.201300584.
46. Sahoo S, Teh TK, He P, et al., 2011, Interface Tissue Engineering: Next Phase in Musculoskeletal Tissue Repair. Ann Acad Med Singapore, 40:245–51.
47. Seidi A, Ramalingam M, Elloumi-Hannachi I, et al., 2011, Gradient Biomaterials for Soft-to-hard Interface Tissue Engineering. Acta Biomater, 7:1441–51. DOI: 10.1016/j.actbio.2011.01.011.
48. Khanarian NT, Jiang J, Wan LQ, et al., 2012, A Hydrogel mineral Composite Scaffold for Osteochondral Interface Tissue Engineering. Tissue Eng Part A, 18:533–45. DOI: 10.1089/ten.tea.2011.0279.
49. Liverani L, Boccaccini AR, 2018, Multi layered Scaffolds for Interface Tissue Engineering Applications. Woodhead Publishing, Sawston, United Kingdom. pp. 107–22.
50. Patel S, Caldwell JM, Doty SB, et al., 2018, Integrating Soft and Hard Tissues Via Interface Tissue Engineering. J Orthop Res, 36:1069–77. DOI: 10.1002/jor.23810.
51. Bracaglia LG, Smith BT, Watson E, et al., 2017, 3D Printing for the Design and Fabrication of Polymer-based Gradient Scaffolds. Acta Biomater, 56:3-13. DOI: 10.1016/j.actbio.2017.03.030