Solvent-based Extrusion 3D Printing for the Fabrication of Tissue Engineering Scaffolds
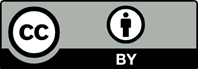
Three-dimensional (3D) printing has been emerging as a new technology for scaffold fabrication to overcome the problems associated with the undesirable microstructure associated with the use of traditional methods. Solvent-based extrusion (SBE) 3D printing is a popular 3D printing method, which enables incorporation of cells during the scaffold printing process. The scaffold can be customized by optimizing the scaffold structure, biomaterial, and cells to mimic the properties of natural tissue. However, several technical challenges prevent SBE 3D printing from translation to clinical use, such as the properties of current biomaterials, the difficulties associated with simultaneous control of multiple biomaterials and cells, and the scaffold-to-scaffold variability of current 3D printed scaffolds. In this review paper, a summary of SBE 3D printing for tissue engineering (TE) is provided. The influences of parameters such as ink biomaterials, ink rheological behavior, crosslinking mechanisms, and printing parameters on scaffold fabrication are considered. The printed scaffold structure, mechanical properties, degradation, and biocompatibility of the scaffolds are summarized. It is believed that a better understanding of the scaffold fabrication process and assessment methods can improve the functionality of SBE-manufactured 3D printed scaffolds.
1. Gross BV, Erkal JL, 2014, Evaluation of 3d Printing and its Potential Impact on Biotechnology and the Chemical Sciences. Anal. Chem., 86:3240–53.
2. Do AV, Khorsand B, Geary SM, et al., 2015, 3D Printing of Scaffolds for Tissue Regeneration Applications. Adv HealthcMater, 4(12):1742–62. DOI: 10.1002/adhm.201500168.
3. Wüst S, Müller R, Hofmann S, 2011, Controlled Positioning of Cells in Biomaterials Approaches towards 3D Tissue Printing. J Funct Biomater, 2(3):119–54. DOI: 10.3390/jfb2030119.
4. Gloria A, Russo T, De Santis R, et al., 2009, 3D Fiber Deposition Technique to Make Multifunctional and Tailormade Scaffolds for Tissue Engineering Applications. J Appl Biomater Biomech, 7(3):141–52.
5. Malda J, Woodfield TB, van der Vloodt F, et al., 2005, The Effect of PEGT/PBT Scaffold Architecture on the Composition of Tissue Engineered Cartilage. Biomaterials, 26(1):63–72. DOI:10.1016/j.biomaterials.2004.02.046.
6. Melchels FP, Tonnarelli B, Olivares AL, et al., 2011, The Influence of the Scaffold Design on the Distribution of Adhering Cells After Perfusion Cell Seeding. Biomaterials, 32(11):2878–84. DOI: 10.1016/j.biomaterials.2011.01.023.
7. Phillippi JA, Miller E, Weiss L, et al., 2008, Microenvironments Engineered by Inkjet Bioprinting Spatially Direct Adult Stem Cells Toward Muscle-and Bone-like Subpopulations. Stem Cells, 26(1):127–34. DOI: 10.1634/stemcells.2007-0520.
8. Woodfield T, Van Blitterswijk CA, De Wijn J, et al., 2005, Polymer Scaffolds Fabricated with Pore-size Gradients as a Model for Studying the Zonal Organization within Tissue engineered Cartilage Constructs. Tissue Eng, 11(9-10):1297–311. DOI: 10.1089/ten.2005.11.1297.
9. Gao, F., Xu Z, Liang Q, et al., 2018, Direct 3D Printing of High Strength Biohybrid Gradient Hydrogel Scaffolds for Efficient Repair of Osteochondral Defect. Adv Funct Mater, 28(13):1706644. DOI: 10.1002/adfm.201706644.
10. Trachtenberg JE, Placone JK, Smith BT, et al., 2017, Extrusion based 3D Printing of Poly (Propylene Fumarate) Scaffolds with Hydroxyapatite Gradients. J Biomater Sci Polym Ed, 28(6):532–54. DOI: 10.1080/09205063.2017.1286184.
11. Hockaday L, Kang KH, Colangelo NW, et al., 2012, Rapid 3D Printing of Anatomically Accurate and Mechanically Heterogeneous Aortic Valve Hydrogel Scaffolds. Biofabrication, 4(3):035005. DOI: 10.1088/1758-5082/4/3/035005.
12. Duan B, Kapetanovic E, Hockaday LA, et al., 2014, Three-dimensional Printed Trileaflet Valve Conduits Using Biological Hydrogels and Human Valve Interstitial Cells. Acta Biomater, 10(5):1836–46. DOI: 10.1016/j.actbio.2013.12.005.
13. Jakus AE, Rutz AL, Jordan SW, et al., 2016, Hyperelastic “Bone”: A Highly Versatile, Growth Factor-free, Osteoregenerative, Scalable, and Surgically Friendly Biomaterial. Sci Transl Med., 8(358):358ra127. DOI:10.1126/scitranslmed.aaf7704.
14. Gonçalves RM, Pereira AC, Pereira IO, et al., 2015, Macrophage Response to Chitosan/poly-(γ-glutamic Acid) Nanoparticles Carrying an Anti-inflammatory Drug. J Mater Sci, 26(4):167. DOI: 10.1007/s10856-015-5496-1.
15. Markstedt K, Mantas A, Tournier I, et al., 2015, 3D Bioprinting Human Chondrocytes with Nanocellulose alginate Bioink for Cartilage Tissue Engineering Applications. Biomacromolecules, 16(5):1489–96. DOI: 10.1021/acs.biomac.5b00188.
16. Sun L, Parker ST, Syoji D, et al., 2012, Direct-Write Assembly of 3D Silk/Hydroxyapatite Scaffolds for Bone Co- Cultures. Adv Healthc Mater, 1(6):729–35. DOI: 10.1002/adhm.201200057.
17. Lee W, Debasitis JC, Lee VK, et al., 2009, Multi-layered Culture of Human Skin Fibroblasts and Keratinocytes Through Three-dimensional Freeform Fabrication. Biomaterials, 30(8):1587–95. DOI: 10.1016/j.biomaterials.2008.12.009.
18. Liu W, Zhang YS, Heinrich MA, et al., 2017, Rapid Continuous Multimaterial Extrusion Bioprinting. Adv Mater, 29(3):1604630.
19. He Y, et al., 2016, Research on the Printability of Hydrogels in 3D Bioprinting. Sci Rep, 6:29977.
20. Lewis JA, Gratson GM, 2004, Direct Writing in Three Dimensions. Mater Today, 7(7-8):32-39.
21. Jang TS, Jung HD, Pan HW, et al., 2018, 3D Printing of Hydrogel Composite Systems: Recent Advances in Technology for Tissue Engineering. Int J Bioprint, 4(1):126.
22. Ozbolat IT., 2016, 3D Bioprinting: Fundamentals, Principles And Applications. Academic Press, London.
23. Derakhshanfar S, Mbeleck R, Xu K, et al., 2018, 3D Bioprinting for Biomedical Devices and Tissue Engineering: A Review of Recent Trends and Advances. Bioact Mater, 3(2):144–56. DOI: 10.1016/j.bioactmat.2017.11.008.
24. Placone JK, Engler AJ, 2018, Recent Advances in Extrusion-Based 3D Printing for Biomedical Applications. Adv HealthcMater, 7(8):e1701161. DOI: 10.1002/adhm.201701161.
25. Schaefer D, Martin I, Jundt G, et al., 2002, Tissue-engineered Composites for the Repair of Large Osteochondral Defects. Arthritis Rheum, 46(9):2524–34.
26. Chen J, Chen H, Li P, et al., 2011, Simultaneous Regeneration of Articular Cartilage and Subchondral Bone in vivo Using MSCs Induced by a Spatially Controlled Gene Delivery System in Bilayered Integrated Scaffolds. Biomaterials, 32(21):4793–805. DOI: 10.1016/j.biomaterials.2011.03.041.
27. Schaefer D, Martin I, Shastri P, et al., 2000, In vitro Generation of Osteochondral Composites. Biomaterials, 21(24):2599–606. DOI: 10.1016/s0142-9612(00)00127-7.
28. Schacht K, Jungst T, Schweinlin M, et al., 2015, Biofabrication of Cell-loaded 3D Spider Silk Constructs. Angew Chem Int Ed, 54(9):2816–20. DOI: 10.1002/anie.201409846.
29. Gao G, Yonezawa T, Hubbell K, et al., 2015, Inkjet-bioprinted Acrylated Peptides and PEG Hydrogel with Human Mesenchymal Stem Cells Promote Robust Bone and Cartilage Formation with Minimal Printhead Clogging. Biotechnol J, 10(10):1568–77. DOI: 10.1002/biot.201400635.
30. Müller M, Becher J, Schnabelrauch M, et al., 2015, Nanostructured Pluronic Hydrogels as Bioinks for 3D Bioprinting. Biofabrication, 7(3):035006. DOI:10.1088/1758-5090/7/3/035006.
31. Wüst S, Godla ME, Müller R, et al., 2014, Tunable Hydrogel Composite with Two-step Processing in Combination with Innovative Hardware Upgrade for Cell-based Three dimensional Bioprinting. Acta Biomater, 10(2):630–40. DOI:10.1016/j.actbio.2013.10.016.
32. Blaeser A, Campos DF, Puster U, et al., 2016, Controlling Shear Stress in 3D Bioprinting is a Key Factor to Balance Printing Resolution and Stem Cell Integrity. Adv HealthcMater, 5(3):326–33. DOI: 10.1002/adhm.201500677.
33. Ghosh S, Parker ST, Wang X, et al., 2008, Direct-write Assembly of Microperiodic Silk Fibroin Scaffolds for Tissue Engineering Applications. Adv Funct Mater, 18(13):1883–9.
DOI: 10.1002/adfm.200800040.
34. Miranda P, Pajares A, Saiz E, et al., 2008, Mechanical Properties of Calcium Phosphate Scaffolds Fabricated by Robocasting. J Biomed Mater Res Part A, 85(1):218–27. DOI: 10.1002/jbm.a.31587.
35. Serra T, Planell JA, Navarro M, 2013, High-resolution PLA based Composite Scaffolds Via 3-D Printing Technology. Acta Biomater, 9(3):5521–30. DOI: 10.1016/j.actbio.2012.10.041.
36. Gonçalves EM, Oliveira FJ, Silva RF, et al., 2016, Three dimensional Printed PCL-hydroxyapatite Scaffolds Filled with CNTs for Bone Cell Growth Stimulation. J Biomed Mater Res Part B, 104(6):1210–9. DOI: 10.1002/jbm.b.33432.
37. Ning L, Guillemot A, Zhao J, et al., 2016, Influence of Flow Behavior of Alginate Cell Suspensions on Cell Viability and Proliferation. Tissue Eng Part C, 22(7):652–62. DOI: 10.1089/ten.tec.2016.0011.
38. Chen DX, Glaser, C, 2019, Extrusion Bioprinting of Scaffolds for Tissue Engineering Applications. Springer, Cham.
39. Dávila JL, d’Ávila MA, 2019, Rheological Evaluation of Laponite/alginate Inks for 3D Extrusion-based Printing. Int J Adv Manuf Technol, 101(1-4):675–86. DOI:10.1007/s00170-018-2876-y.
40. Liu S, Li L, 2016, Recoverable and Self-healing Double Network Hydrogel Based on κ-Carrageenan. ACS Appl Mater Interfaces, 8(43):29749–58. DOI: 10.1021/acsami.6b11363.
41. Freeman FE, Kelly DJ, 2017, Tuning Alginate Bioink Stiffness and Composition for Controlled Growth Factor Delivery and to Spatially Direct MSC fate Within Bioprinted Tissues. Sci Rep, 7(1):17042. DOI: 10.1038/s41598-017-17286-1.
42. Chung JH, Naficy S, Yue Z, et al., 2013, Bio-ink Properties and Printability for Extrusion Printing Living Cells. Biomater Sci, 1(7):763–73. DOI: 10.1039/c3bm00012e.
43. Donderwinkel I, van Hest JC, Cameron NR, 2017, Bio-inks for 3D Bioprinting: Recent Advances and Future Prospects. Polym Chem, 8(31):4451–71. DOI: 10.1039/c7py00826k.
44. Li H, Tan C, Li L, 2018, Review of 3D Printable Hydrogels and Constructs. Mater Des, 159:20–38. DOI: 10.1016/j. matdes.2018.08.023.
45. Ouyang L, Yao R, Zhao Y, et al., 2016, Effect of Bioink Properties on Printability and Cell Viability for 3D Bioplotting of Embryonic Stem Cells. Biofabrication, 8(3):035020. DOI: 10.1088/1758-5090/8/3/035020.
46. Knowlton S, Yenilmez B, Anand S, et al., 2017, Photo crosslinking-based Bioprinting: Examining Crosslinking Schemes. Bioprinting, 5:10–8. DOI: 10.1016/j.bprint.2017.03.001.
47. Schuurman W, Levett PA, Pot MW, et al., 2013, Gelatinmethacrylamide Hydrogels as Potential Biomaterials for Fabrication of Tissue-engineered Cartilage Constructs. Macromol Biosci, 13(5):551–61. DOI: 10.1002/mabi.201200471.
48. Pescosolido L, Schuurman W, Malda J, et al., 2011, Hyaluronic Acid and Dextran-based Semi-IPN Hydrogels as Biomaterials for Bioprinting. Biomacromolecules, 12(5):1831–8. DOI: 10.1021/bm200178w.
49. Morrison F, 2001, Understanding Rheology. Oxford University Press, New York.
50. You F, Wu X, Chen X, 2017, 3D Printing of Porous Alginate/gelatin Hydrogel Scaffolds and Their Mechanical Property Characterization. Int J Polym Mater Polym Biomater, 66(6):299–306. DOI: 10.1080/00914037.2016.1201830.
51. Srivas PK, Kapat K, Dadhich P, et al., 2017, Osseointegration Assessment of Extrusion Printed Ti6Al4V Scaffold Towards Accelerated Skeletal Defect Healing Via Tissue In-growth. Bioprinting, 6:8–17. DOI: 10.1016/j.bprint.2017.04.002.
52. Campos Marin A, Lacroix D, 2015, The Inter-sample Structural Variability of Regular Tissue-engineered Scaffolds Significantly Affects the Micromechanical Local Cell Environment. Interface Focus, 5(2):20140097. DOI 10.1098/rsfs.2014.0097.
53. Colosi C, Shin SR, Manoharan V, et al., 2016, Microfluidic Bioprinting of Heterogeneous 3D Tissue Constructs Using Low-viscosity Bioink. Adv Mater, 28(4):677–84. DOI:10.1002/adma.201503310.
54. Jeon O, Song SJ, Lee KJ, et al., 2007, Mechanical Properties and Degradation Behaviors of Hyaluronic Acid Hydrogels Cross-linked at Various Cross-linking Densities. Carbohydr. Polym, 70(3):251–7. DOI: 10.1016/j.carbpol.2007.04.002.
55. Jia W, Gungor-Ozkerim PS, Zhang YS, et al., 2016, Direct 3D Bioprinting of Perfusable Vascular Constructs Using a Blend Bioink. Biomaterials, 106:58–68. DOI: 10.1016/j. biomaterials.2016.07.038.
56. Duan B, Hockaday LA, Kang KH, et al., 2013, 3D Bioprinting of Heterogeneous Aortic Valve Conduits with Alginate/ gelatin Hydrogels. J Biomed Mater Res, 101(5):1255–64. DOI: 10.1002/jbm.a.34420.
57. Rutz AL, Hyland KE, Jakus AE, et al., 2015, A Multimaterial Bioink Method for 3D Printing Tunable, Cell-Compatible Hydrogels. Adv Mater, 27(9):1607–14. DOI: 10.1002/ adma.201405076.
58. Wu Z, Su X, Xu Y, et al., 2016, Bioprinting Three-dimensional Cell-laden Tissue Constructs with Controllable Degradation. Sci Rep, 6:24474. DOI: 10.1038/srep24474.
59. Ragaert K, Maeyaert G, Martins CI, et al., 2014, Bulk Compounding of PCL-PEO Blends for 3D Plotting of Scaffolds for Cardiovascular Tissue Engineering. J Mater Sci Eng, 3(1):136. DOI: 10.4172/2169-0022.1000136.
60. Remya K, Chandran S, Mani S, et al., 2018, Hybrid Polycaprolactone/Polyethylene Oxide Scaffolds with Tunable Fiber Surface Morphology, Improved Hydrophilicity and Biodegradability for Bone Tissue Engineering Applications. J Biomater Sci, 29(12):1444–62. DOI: 10.1080/09205063.2018.1465664.
61. Lyons JG, Blackie P, Higginbotham CL, 2008, The Significance of Variation in Extrusion Speeds and Temperatures on a PEO/PCL Blend Based Matrix for Oral Drug Delivery. Int J Pharm, 351(1–2):201–8. DOI: 10.1016/j.ijpharm.2007.09.041.
62. Kuo M, Yen S, 2002, The Process of Electrochemical Deposited Hydroxyapatite Coatings on Biomedical Titanium at Room Temperature. Mater Sci Eng C, 20(1):153–60. DOI:10.1016/s0928-4931(02)00026-7.
63. Murugan R, Ramakrishna S, 2005, Development of Nanocomposites for Bone Grafting. Compos Sci Technol, 65(15-16):2385–406.
64. Kolan K, Liu Y, Baldridge J, et al., 2017, Solvent Based 3D Printing of Biopolymer/Bioactive Glass Composite and Hydrogel for Tissue Engineering Applications. Procedia CIRP, 65:38–43. DOI: 10.1016/j.procir.2017.04.022.
65. Zheng Y, Gu X, Witte F, 2014, Biodegradable Metals. Mater Sci Eng, 77:1–34.
66. Wong HM, Wu S, Chu PK, et al., 2013, Low-modulus Mg/PCL Hybrid Bone Substitute for Osteoporotic Fracture Fixation. Biomaterials, 34(29):7016–32. DOI: 10.1016/j. biomaterials.2013.05.062.
67. Ning L, Chen X, 2017, A Brief Review of Extrusion-based Tissue Scaffold Bio-printing. Biotechnol J, 12(8):1600671. DOI: 10.1002/biot.201600671.
68. Marin AC, 2016, In silico Study of the Mechanisms of Cell Deposition into 3D Rapid Prototyping Scaffolds Under in vitro Hydrodynamic Conditions. Thesis. University of Sheffield.
69. Melchels FP, Bertoldi K, Gabbrielli R, et al., 2010, Mathematically Defined Tissue Engineering Scaffold Architectures Prepared by Stereolithography. Biomaterials, 31(27):6909–16. DOI: 10.1016/j.biomaterials.2010.05.068.
70. Grayson WL, Fröhlich M, Yeager K, et al., 2010, Engineering Anatomically Shaped Human Bone Grafts. Proc Natl Acad Sci, 107(8):3299–304. DOI: 10.1073/pnas.0905439106