Effects of DNA methylation and gene expression on rats with protein malnutrition in early life
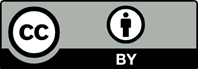
Although the mechanisms underlying how malnutrition in early life affects the susceptibility to diseases later in life remain unclear, there is considerable interest in the potential role of DNA methylation in the intrauterine programming of diseases. In this study, digital gene expression profiles were used to analyze changes in gene expression of protein-restricted rats early in life, while intergenerational rat models were used to explore differences in whole blood genomic DNA methylation in an environment stimulated by maternal protein intervention. Nine rats were randomly divided into early-life low-protein group (LPE), fetal low-protein group (LPF), and normal control group (CON). The LPE group was fed a low-protein diet on the 1st day of pregnancy until the end of lactation. The LPF group was given low-protein diet during pregnancy. The CON group was given a 20% protein diet from the 1st day of pregnancy. Total mRNA was extracted from the sacrificed rats at the 48th week. The number of differentially expressed genes for LPE versus CON, LPF versus CON, and LPF versus LPE was 178, 223, and 302, respectively. Comparing LPE versus CON and LPF versus CON, the upregulated genes common to the two groups were Gimap-9, Serinc-4, Dnah-2, Sf3b-5, and Sat-2, and the downregulated genes were Ppp1r-3. Comparing LPF versus CON and LPF versus LPE, the upregulated genes were Mgat2 and Cars, and the downregulated genes were Ddx28 and Slc12a9. The differentially expressed genes were mainly related to cell metabolism, immune response, signaling pathway, endocrine metabolism, stress response, ATP binding, and other functions. Early-life protein malnutrition affects gene expression of rat offspring and involves multiple aspects of growth and development, with different stages of early-life malnutrition leading to altered DNA methylation expression of corresponding genes, mainly in mitochondrial genes.
Sciascia QL, Prehn C, Adamski J, et al., 2021, The effect of dietary protein imbalance during pregnancy on the growth, metabolism and circulatory metabolome of neonatal and weaned juvenile porcine offspring. Nutrients, 13(9): 3286. https://doi.org/10.3390/nu13093286
Kanakis I, Alameddine M, Folkes L, et al., 2021, Small- RNA sequencing reveals altered skeletal muscle microRNAs and snoRNAs signatures in weanling male offspring from mouse dams fed a low protein diet during lactation. Cells, 10(5): 1166. https://doi.org/10.3390/cells10051166
Agnoux AM, El Ghaziri A, Moyon T, et al., 2018, Maternal protein restriction during lactation induces early and lasting plasma metabolomic and hepatic lipidomic signatures of the offspring in a rodent programming model. J Nutr Biochem, 55: 124–141. https://doi.org/10.1016/j.jnutbio.2017.11.009
Marin BK, de Lima Reis SR, de Fitima Silva Ramalho A, et al., 2019, Protein restriction in early life increases intracellular calcium and insulin secretion, but does not alter expression of SNARE proteins during pregnancy. Exp Physiol, 104(7): 1029–1037. https://doi.org/10.1113/EP087045
Loche E, Ozanne SE, 2016, Early nutrition, epigenetics, and cardiovascular disease. Curr Opin Lipidol, 27(5): 449–458. https://doi.org/10.1097/MOL.0000000000000338
De Vasconcelos Barros MA, de Brito Alves JL, Barros RGN, et al., 2020, Effects of maternal protein restriction on central and peripheral renin-angiotensin systems in male rat offspring. Life Sci, 263: 118574. https://doi.org/10.1016/j.lfs.2020.118574
Fesser EA, Gianatiempo O, Berardino BG, et al., 2021, Limited contextual memory and transcriptional dysregulation in the medial prefrontal cortex of mice exposed to early protein malnutrition are intergenerationally transmitted. J Psychiatr Res, 139: 139–149. https://doi.org/10.1016/j.jpsychires.2021.05.041
Fernandez-Twinn DS, Constância M, Ozanne SE, 2015, Intergenerational epigenetic inheritance in models of developmental programming of adult disease. Semin Cell Dev Biol, 43: 85–95. https://doi.org/10.1016/j.semcdb.2015.06.006
Yuasa K, Kondo T, Nagai H, et al., 2016, Maternal protein restriction that does not have an influence on the birthweight of the offspring induces morphological changes in kidneys reminiscent of phenotypes exhibited by intrauterine growth retardation rats. Cong Anom, 56(2): 79–85. https://doi.org/10.1111/cga.12143
Miyoshi M, Sato M, Saito K, et al., 2018, Maternal protein restriction alters the renal Ptger1 DNA methylation state in SHRSP offspring. Nutrients, 10(10): 1436. https://doi.org/10.3390/nu10101436
Zhang D, Liu X, Yang M, et al., 2018, Maternal protein restriction induces alterations in Nkx2. 2 expression in pancreatic cells of intrauterine growth restriction rats. Int J Clin Exp Med, 11(12): 13468–13479.
De Arruda EHP, da Silva GLV, da Rosa-Santos CA, et al., 2020, Protein restriction during pregnancy impairs intra-islet GLP-1 and the expansion of cells of i. Mol Cell Endocrinol, 518: 110977. https://doi.org/10.1016/j.mce.2020.110977
Anvar Z, Chakchouk I, Demond H, et al., 2021, DNA methylation dynamics in the female germline and maternal-effect mutations that disrupt genomic imprinting. Genes, 12(8): 1214. https://doi.org/10.3390/genes12081214
Kim J, Choi A, Kwon YH, 2020, Maternal protein restriction altered insulin resistance and inflammation-associated gene expression in adipose tissue of young adult mouse offspring in response to a high-fat diet. Nutrients, 12(4): 1103. https://doi.org/10.3390/nu12041103
Boubred F, Daniel L, Buffat C, et al., 2009, Early postnatal overfeeding induces early chronic renal dysfunction in adult male rats. Am J Physiol Renal Physiol, 297(4): F943. https://doi.org/10.1152/ajprenal.90704.2008
Pond WG, Mersmann HJ, Yen JT, 1985, Severe feed restriction of pregnant swine and rats: Effects on postweaning growth and body composition of progeny. J Nutr, 115(2): 179–189. https://doi.org/10.1093/jn/115.2.179
Tomi M, Zhao Y, Thamotharan S, et al., 2013, Early life nutrient restriction impairs blood-brain metabolic profile and neurobehavior predisposing to Alzheimer’s disease with aging. Brain Res, 1495:61–75. https://doi.org/10.1016/j.brainres.2012.11.050
Ripsin C, 2009, The metabolic syndrome: Underdiagnosed and undertreated. South Med J, 102(12): 1194–1195. https://doi.org/10.1097/SMJ.0b013e3181c03173
Haque M, Starr LM, Koski KG, et al., 2018, Differential expression of genes in fetal brain as a consequence of maternal protein deficiency and nematode infection. Int J Parasitol, 48(1): 51–58. https://doi.org/10.1016/j.ijpara.2017.07.005
De Sousa SM, Braz GRF, Freitas CM, et al., 2018, Oxidative injuries induced by maternal low-protein diet in female brainstem. Nutr Neurosci, 21(8): 580–588. https://doi.org/10.1080/1028415X.2017.1325974
Danson AF, Marzi SJ, Lowe R, et al., 2018, Early life diet conditions the molecular response to post-weaning protein restriction in the mouse. BMC Biol, 16(1): 51. https://doi.org/10.1186/s12915-018-0516-5
Rinaldi JC, Santos SAA, Colombelli KT, et al., 2018, Maternal protein malnutrition: Effects on prostate development and adult disease. J Dev Orig Health Dis, 9(4): 361–372. https://doi.org/10.1017/S2040174418000168
Odhiambo JF, Pankey CL, Ghnenis AB, et al., 2020, A review of maternal nutrition during pregnancy and impact on the offspring through development: evidence from animal models of over-and undernutrition. Int J Environ Res Public Health, 17(18): 6926. https://doi.org/10.3390/ijerph17186926
Ferroni NM, Berardino BG, Belluscio LM, et al., 2020, Perinatal protein malnutrition induces the emergence of enduring effects and age-related impairment behaviors, increasing the death risk in a mouse model. Nutr Neurosci, 2020: 1–14. https://doi.org/10.1080/1028415X.2020.1829343
Greenberg MVC, Bourcurcg D, 2019, The diverse roles of DNA methylation in mammalian development and disease. Nat Rev Mol Cell Biol, 20(10): 590–607. https://doi.org/10.1038/s41580-019-0159-6
Nätt D, Barchiesi R, Murad J, et al., 2017, Perinatal malnutrition leads to sexually dimorphic behavioral responses with associated epigenetic changes in the mouse brain. Sci Rep, 7(1): 1–14. https://doi.org/10.1038/s41598-017-10803-2
Jin Z, Liu Y, 2018, DNA methylation in human diseases. Genes Dis, 5(1): 1–8. https://doi.org/10.1016/j.gendis.2018.01.002
Pan Y, Liu G, Zhou F, et al., 2018, DNA methylation profiles in cancer diagnosis and therapeutics. Clin Exp Med, 18(1): 1–14. https://doi.org/10.1007/s10238-017-0467-0
Herttuala S, Baker AH, 2017, Cardiovascular gene therapy: Past, present, and future. Mol Ther, 25: 1095–1106. https://doi.org/10.1016/j.ymthe.2017.03.027
Crispi F, Miranda J, Gratacós E, 2018, Long-term cardiovascular consequences of fetal growth restriction: biology, clinical implications, andopportunities for prevention of adult disease. Am J Obst Gynecol, 218(2): S869–S879. https://doi.org/10.1016/j.ajog.2017.12.012
Magalhães ESD, Méio MDB, Moreira MEL, 2019, Hormonal biomarkers for evaluating the impact of fetal growth restriction on the development of chronic adult disease. Rev Bras Ginecol Obst, 41: 256–263. https://doi.org/10.1055/s-0039-1683904
Campisano S, La Colla A, Echarte SM, et al., 2019, Interplay between early-life malnutrition, epigenetic modulation of the immune function and liver diseases. Nutr Res Rev, 32: 128–145. https://doi.org/10.1017/S0954422418000239
May L, Newton ER, 2017, Adaptation of maternal-fetal physiology to exercise in pregnancy: The basis of guidelines for physical activity in pregnancy. Clin Med Insights Womens Health, 10: 1179562X17693224. https://doi.org/10.1177/1179562X17693224
Wang LM, Chen ZH, Zhang M, et al., 2019, Study of the prevalence and disease burden of chronic disease in the elderly in China. Zhonghua Liu Xing Bing Xue Za Zhi, 40(3): 277–283. https://doi.org/10.3760/cma.j.issn.0254-6450.2019.03.005
Seymour DK, Gaut BS, 2020, Phylogenetic shifts in gene body methylation correlate with gene expression and reflect trait conservation. Mol Biol Evol, 37(1): 31–43.https://doi.org/10.1093/molbev/msz195
Oke SL, Gurjeev S, Hardy DB, 2020, Perinatal protein restriction with postnatal catch-up growth leads to elevated p66Shc and mitochondrial dysfunction in the adult rat liver. Reproduction, 159: 27–39. https://doi.org/10.1530/REP-19-0188
Wu Y, Cheng Z, Bai Y, et al., 2019, Epigenetic mechanisms of maternal dietary protein and amino acids affecting growth and development of offspring. Curr Protein Pept Sci, 20: 727–735. https://doi.org/10.2174/1389203720666190125110150
Horvath S, Raj K, 2018, DNA methylation-based biomarkers and the epigenetic clock theory of ageing. Nat Rev Genet, 19: 371–384. https://doi.org/10.1038/s41576-018-0004-3
Dugué PA, Bassett JK, Joo JE, et al., 2018, Association of DNA methylation-based biological age with health risk factors, and overall and cause-specific mortality. Am J Epidemiol, 187(3): 529–538. https://doi.org/10.1093/aje/kwx291
Andreucci CB, Zanardi DT, Cecatti JG, et al., 2021, Longterm consequences of severe maternal morbidity on infant growth and development. Matern Child Health J, 25(1): 487–496. https://doi.org/10.1007/s10995-020-03070-7
Armengaud JB, Yzydorczyk C, Siddeek B, et al., 2020, Intrauterine growth restriction: Clinical consequences on health and disease at adulthood. Reprod Toxicol, 99: 168–176. https://doi.org/10.1016/j.reprotox.2020.10.005
Joung KE, Lee J, Kim JH, 2020, Long-term metabolic consequences of intrauterine growth restriction. Curr Pediatr Rep, 8(3): 45–55.
Ehrlich M, 2019, DNA hypermethylation in disease: Mechanisms and clinical relevance. Epigenetics, 14(7539): 1–23. https://doi.org/10.1080/15592294.2019.1638701
Tang L, 2015, Effect of Extrauterine Growth Restriction on Pulmonary Vasculature in First and Second Generation: Altered Epigenetic Programming. China: Zhejiang University.
Kuang X, 2012, Study of the relationship between WTl and IGF2 DNA methylation and kidney development and function in intrauterine growth restriction rat. China: Fudan University.
Chen X, Wang Y, Chen G, et al., 2014, Expression and significance of phosphatidylinositol-3 kinase p110α in the hippocampus of rats born with early malnutrition. Chin J Gerontol, 34(5):1299–1302.
Da Costa NM, Visoni SB, Dos Santos IL, et al., 2016, Maternal protein restriction during lactation modulated the expression and activity of rat offspring hepatic CYP1A1, CYP1A2, CYP2B1, CYP2B2, and CYP2E1 during development. Brazil J Med Biol Res, 49(11): e5238. https://doi.org/10.1590/1414-431X20165238
Chellappan DK, Sivam NS, Teoh KX, et al., 2018, Gene therapy and Type 1 diabetes mellitus. Biomed Pharmacother, 108: 1188–1200. https://doi.org/10.1016/j.biopha.2018.09.138
Lin R, Ye Z, Wang H, et al., Chronic diseases and health monitoring big data: A survey. IEEE Rev Biomed Eng, 11: 275–288. https://doi.org/10.1109/RBME.2018.2829704
Kirigiti MA, Frazee T, Bennett BJ, et al., 2020, Effects of pre and postnatal protein restriction on maternal and offspring metabolism in the nonhuman primate. Am J Physiol Regul Integr Comp Physiol, 318(440): R929–R939. https://doi.org/10.1152/ajpregu.00150.2019
Ratsika A, Codagnone MC, O’Mahony S, et al., 2021, Priming for life: Early life nutrition and the microbiota-gut-brain axis. Nutrients, 13(2): 423. https://doi.org/10.3390/nu13020423