Swelling compensation of engineered vasculature fabricated by additive manufacturing and sacrifice-based technique using thermoresponsive hydrogel
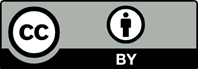
Engineered vasculature is widely employed to maintain the cell viability within in vitro tissues. A variety of fabrication techniques for engineered vasculature have been explored, with combination of additive manufacturing with a sacrifice-based technique being the most common approach. However, the size deformation of vasculature caused by the swelling of sacrificial materials remains unaddressed. In this study, Pluronic F-127 (PF-127), the most widely used sacrificial material, was employed to study the deformation of the vasculature. Then, a thermoresponsive hydrogel comprising poly(N-isopropylacrylamide) (PNIPAM) and gelatin methacrylate (GelMA) was used to induce volume shrinkage at 37°C to compensate for the deformation of vasculature caused by the swelling of a three-dimensional (3D)- printed sacrificial template, and to generate vasculature of a smaller size than that after deformation. Our results showed that the vasculature diameter increased after the sacrificial template was removed, whereas it decreased to the designed diameter after the volume shrinkage. Human umbilical vein endothelial cells (HUVECs) formed an endothelial monolayer in the engineered vasculature. Osteosarcoma cells (OCs) were loaded into a hierarchical vasculature within the thermoresponsive hydrogel to investigate the interaction between HUVECs and OCs. New blood vessel infiltration was observed within the lumen of the engineered vasculature after in vivo subcutaneous implantation for 4 weeks. In addition, engineered vasculature was implanted in a rat ischemia model to further study the function of engineered vasculature for blood vessel infiltration. This study presents a small method aiming to accurately create engineered vasculature by additive manufacturing and a sacrificebased technique.
Dumas SJ, Meta E, Borri M, et al., 2021, Phenotypic diversity and metabolic specialization of renal endothelial cells. Nat Rev Nephrol, 17(7): 441–464. https://doi.org/10.1038/s41581-021-00411-9.
Liu X, Wang X, Zhang L, et al., 2021, 3D liver tissue model with branched vascular networks by multimaterial bioprinting. Adv Healthc Mater, 10(23): 2101405. https://doi.org/10.1002/adhm.202101405.
Zhu Y, Kong B, Liu R, et al., 2022, Developing biomedical engineering technologies for reproductive medicine. Smart Med, 1(1): e20220006. https://doi.org/10.1002/smmd.20220006.
Fu Z, Zhuang Y, Cui J, et al., 2022, Development and challenges of cells- and materials-based tooth regeneration. Eng Reg, 3(2): 163–181. https://doi.org/10.1016/j.engreg.2022.04.003.
Gao Y, Ma Q, 2022, Bacterial infection microenvironment‐responsive porous microspheres by microfluidics for promoting anti‐infective therapy. Smart Med, 1(1): e2022001. https://doi.org/10.1002/smmd.20220012.
Sarker MD, Naghieh S, Sharma NK, et al., 2018, 3D biofabrication of vascular networks for tissue regeneration: a report on recent advances. J Pharm Anal, 8(5): 277–296. https://doi.org/10.1016/j.jpha.2018.08.005.
Nie J, Gao Q, Wang Y, et al., 2018, Vessel-on-a-chip with hydrogel-based microfluidics. Small, 14(45): 1802368. https://doi.org/10.1002/smll.201802368.
John JV, McCarthy A, Wang H, et al., 2021, Freeze‐casting with 3D‐printed templates creates anisotropic microchannels and patterned macrochannels within biomimetic nanofiber aerogels for rapid cellular infiltration. Adv Healthc Mater, 10(12): 2100238. https://doi.org/10.1002/adhm.202100238.
Zhang B, Radisic M, 2020, Organ-level vascularization: The Mars mission of bioengineering. J Thorac Cardiovasc Surg, 159(5): 2003–2007. https://doi.org/10.1016/j.jtcvs.2019.08.128.
Kinstlinger IS, Saxton SH, Calderon GA, et al., 2020, Generation of model tissues with dendritic vascular networks via sacrificial laser-sintered carbohydrate templates. Nat Biomed Eng, 4(9): 916–932. https://doi.org/10.1038/s41551-020-0566-1.
Cui H, Esworthy T, Zhou X, et al., 2020, Engineering a novel 3D printed vascularized tissue model for investigating breast cancer metastasis to bone. Adv Healthc Mater, 9(15): 1900924. https://doi.org/10.1002/adhm.201900924.
Lee B, Kim S, Ko J, et al., 2022, 3D micromesh-based hybrid bioprinting: Multidimensional liquid patterning for 3D microtissue engineering. NPG Asia Mater, 14: 6. https://doi.org/10.1038/s41427-022-00355-x.
Loterie D, Delrot P, Moser C, 2020, High-resolution tomographic volumetric additive manufacturing. Nat Commun, 11: 852. https://doi.org/10.1038/s41467-020-14630-4.
Thomas A, Orellano I, Lam T, et al., 2020, Vascular bioprinting with enzymatically degradable bioinks via multi-material projection-based stereolithography. Acta Biomater, 117: 121–132. https://doi.org/10.1016/j.actbio.2020.09.033.
Grigoryan B, Paulsen SJ, Corbett DC, et al., 2019, Multivascular networks and functional intravascular topologies within biocompatible hydrogels. Science, 364(6439): 458–464. https://www.science.org/doi/10.1126/science.aav9750.
Szklanny AA, Machour M, Redenski I, et al., 2021, 3D bioprinting of engineered tissue flaps with hierarchical vessel networks (VesselNet) for direct host‐to‐implant perfusion. Adv Mater, 33(42): 2102661. https://doi.org/10.1002/adma.202102661.
Wu W, DeConinck A, Lewis JA, 2011, Omnidirectional printing of 3D microvascular networks. Adv Mater, 23(24): H178–H183. https://doi.org/10.1002/adma.201004625.
Kolesky DB, Truby RL, Gladman AS, et al., 2014, 3D bioprinting of vascularized, heterogeneous cell‐laden tissue constructs. Adv Mater, 26(19): 3124–3130. https://doi.org/10.1002/adma.201305506.
Kolesky DB, Homan KA, Skylar-Scott MA, et al., 2016, Three-dimensional bioprinting of thick vascularized tissues. Proc Natl Acad Sci, 113(12): 3179–3184. https://www.pnas.org/lookup/suppl/doi:10.1073/ pnas.1521342113/-/DCSupplemental.
Lin NYC, Homan KA, Robinson SS, et al., 2019, Renal reabsorption in 3D vascularized proximal tubule models. Proc Natl Acad Sci, 116(12): 5399–5404. https://www.pnas.org/lookup/suppl/doi:10.1073/ pnas.1815208116/-/DCSupplemental.
Neufeld L, Yeini E, Reisman N, et al., 2021, Microengineered perfusable 3D-bioprinted glioblastoma model for in vivo mimicry of tumor microenvironment. Sci Adv, 7(14): eabi9119. https://www.science.org/doi/10.1126/sciadv.abi9119.
Wu C-J, Gaharwar AK, Chan BK, et al., 2011, Mechanically tough pluronic F127/laponite nanocomposite hydrogels from covalently and physically cross-linked networks. Macromolecules, 44(20): 8215–8224. https://doi.org/10.1021/ma200562k.
Hu M, Dailamy A, Lei XY, et al., 2018, Facile engineering of long‐term culturable ex vivo vascularized tissues using biologically derived matrices. Adv Healthc Mater, 7(23): 1800845. https://doi.org/10.1002/adhm.201800845.
Tocchio A, Tamplenizza M, Martello F, et al., 2015, Versatile fabrication of vascularizable scaffolds for large tissue engineering in bioreactor. Biomaterials, 45: 124–131. http://dx.doi.org/10.1016/j.biomaterials.2014.12.031.
Li S, Liu Y-Y, Liu L-J, et al., 2016, A versatile method for fabricating tissue engineering scaffolds with a three-dimensional channel for prevasculature networks. ACS Appl Mater Interfaces, 8(38): 25096–25103. https://doi.org/10.1021/acsami.6b07725.
Yang X, Zhang C, Deng D, et al., 2022, Multiple stimuli-responsive MXene-based hydrogel as intelligent drug delivery carriers for deep chronic wound healing. Small, 18(5): 2104368. https://doi.org/10.1002/smll.202104368.
Zhang Z, Chen Z, Wang Y, et al., 2019, Bioinspired bilayer structural color hydrogel actuator with multienvironment responsiveness and survivability. Small Methods, 3(12): 1900519. https://doi.org/10.1002/smtd.201900519.
Dabiri SMH, Samiei E, Shojaei S, et al., 2021, Multifunctional thermoresponsive microcarriers for high‐throughput cell culture and enzyme‐free cell harvesting. Small, 17(44): 2103192. https://doi.org/10.1002/smll.202103192.
Cui H, Wang W, Shi L, et al., 2020, Superwettable surface engineering in controlling cell adhesion for emerging bioapplications. Small Methods, 4(12): 2000573. https://doi.org/10.1002/smtd.202000573.
Liu M, Wu C, Ke L, et al., 2021, Emerging biomaterials‐based strategies for inhibiting vasculature function in cancer therapy. Small Methods, 5(7): 2100347. https://doi.org/10.1002/smtd.202100347.
Downs FG, Lunn DJ, Booth MJ, et al., 2020, Multi-responsive hydrogel structures from patterned droplet networks. Nat Chem, 12: 363–371. https://doi.org/10.1038/s41557-020-0444-1.
Kan X, Wu C, Wen L, et al., 2020, Biomimetic nanochannels: From fabrication principles to theoretical insights. Small Methods, 6(4): 2101255. https://doi.org/10.1002/smtd.202101255.
Wang X, Yu Y, Yang C, et al., 2021, Microfluidic 3D printing responsive scaffolds with biomimetic enrichment channels for bone regeneration. Adv Funct Mater, 31(40): 2105190. https://doi.org/10.1002/adfm.202105190.
Li S, Wang W, Li W, et al., 2021, Fabrication of thermoresponsive hydrogel scaffolds with engineered microscale vasculatures. Adv Funct Mater, 31(27): 2102685. https://doi.org/10.1002/adfm.202102685.
Luo Z, Che J, Sun L, et al., 2021, Microfluidic electrospray photo-crosslinkable κ-Carrageenan microparticles for wound healing. Eng Reg, 2: 257–262. https://doi.org/10.1016/j.engreg.2021.10.002.
Layek RK, Uddin ME, Kim NH, et al., 2017, Noncovalent functionalization of reduced graphene oxide with pluronic F127 and its nanocomposites with gum arabic. Compos B Eng, 128: 155–163. https://doi.org/10.1016/j.compositesb.2017.07.010.
Schmidt S, Zeiser M, Hellweg T, et al., 2010, Adhesion and mechanical properties of PNIPAM microgel films and their potential use as switchable cell culture substrates. Adv Funct Mater, 20: 3235–3243. https://doi.org/10.1002/adfm.201000730.
Zhu CH, Lu Y, Peng J, et al., 2012, Photothermally sensitive poly(N-isopropylacrylamide)/graphene oxide nanocomposite hydrogels as remote light-controlled liquid microvalves. Adv Funct Mater, 22(19): 4017–4022. https://doi.org/10.1002/adfm.201201020.
Wu L, Pei X, Zhang B, et al., 2022, 3D-printed HAp bone regeneration scaffolds enable nano-scale manipulation of cellular mechanotransduction signals. J Chem Eng, 455: 140699. https://doi.org/10.1016/j.cej.2022.140699.
Chung TW, Liu DZ, Wang SY, et al., 2003, Enhancement of the growth of human endothelial cells by surface roughness at nanometer scale. Biomaterials, 24(25): 4655–4661. https://doi.org/10.1016/s0142-9612(03)00361-2.
Cooperstein MA, Canavan HE, 2013, Assessment of cytotoxicity of (N-isopropyl acrylamide) and poly(N-isopropyl acrylamide)-coated surfaces. Biointerphases, 8(1): 19. https://doi.org/10.1186/1559-4106-8-19.
Xu L, Varkey M, Jorgensen A, et al., 2020, Bioprinting small diameter blood vessel constructs with an endothelial and smooth muscle cell bilayer in a single step. Biofabrication, 12(4): 045012. https://doi.org/10.1088/1758-5090/aba2b6.
Xie M, Gao Q, Zhao H, et al., 2019, Electro-assisted bioprinting of low-concentration GelMA microdroplets. Small, 15(4): 1804216. https://doi.org/10.1002/smll.201804216.
Song P, Li M, Zhang B, et al., 2022, DLP fabricating of precision GelMA/HAp porous composite scaffold for bone tissue engineering application. Compos B Eng, 244: 110163. https://doi.org/10.1016/j.compositesb.2022.110163.