Osteoconductivity of bone substitutes with filament-based microarchitectures: Influence of directionality, filament dimension, and distance
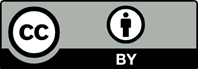
Additive manufacturing can be applied to produce personalized bone substitutes. At present, the major three-dimensional (3D) printing methodology relies on filament extrusion. In bioprinting, the extruded filament consists mainly of hydrogels, in which growth factors and cells are embedded. In this study, we used a lithography-based 3D printing methodology to mimic filament-based microarchitectures by varying the filament dimension and the distance between the filaments. In the first set of scaffolds, all filaments were aligned toward bone ingrowth direction. In a second set of scaffolds, which were derived from the identical microarchitecture but tilted by 90°, only 50% of the filaments were in line with the bone ingrowth direction. Testing of all tricalcium phosphate-based constructs for osteoconduction and bone regeneration was performed in a rabbit calvarial defect model. The results revealed that if all filaments are in line with the direction of bone ingrowth, filament size and distance (0.40–1.25 mm) had no significant influence on defect bridging. However, with 50% of filaments aligned, osteoconductivity declined significantly with an increase in filament dimension and distance. Therefore, for filament-based 3D- or bio-printed bone substitutes, the distance between the filaments should be 0.40 to 0.50 mm irrespective of the direction of bone ingrowth or up to 0.83 mm if perfectly aligned to it.
1. Weber FE, 2019, Reconsidering osteoconduction in the era of additive manufacturing. Tissue Eng Part B Rev, 25(5): 375–386. https://doi.org/10.1089/ten.TEB.2019.0047
2. de Wild M, Schumacher R, Mayer K, et al., 2013, Bone regeneration by the osteoconductivity of porous titanium implants manufactured by selective laser melting: A histological and micro computed tomography study in the rabbit. Tissue Eng Part A, 19(23–24): 2645–2654. https://doi.org/10.1089/ten.TEA.2012.0753
3. Sears NA, Seshadri DR, Dhavalikar PS, et al., 2016, A review of three-dimensional printing in tissue engineering. Tissue Eng Part B Rev, 22(4): 298–310. https://doi.org/10.1089/ten.TEB.2015.0464
4. Brunello G, Sivolella S, Meneghello R, et al., 2016, Powder-based 3D printing for bone tissue engineering. Biotechnol Adv, 34(5): 740–753. https://doi.org/10.1016/j.biotechadv.2016.03.009
5. Sachs E, Cima M, Cornie J, 1990, Three-dimensional printing: Rapid tooling and prototypes directly from a CAD model. CIRP Ann Manuf Technol, 39(1): 201–204. http://dx.doi.org/10.1016/S0007-8506(07)61035-X
6. Butscher A, Bohner M, Doebelin N, et al., 2013, New depowdering-friendly designs for three-dimensional printing of calcium phosphate bone substitutes. Acta Biomater, 9(11): 9149–9158. http://dx.doi.org/10.1016/j.actbio.2013.07.019
7. Butscher A, Bohner M, Roth C, et al., 2012, Printability of calcium phosphate powders for three-dimensional printing of tissue engineering scaffolds. Acta Biomater, 8: 373–385.
8. Karageorgiou V, Kaplan D, 2005, Porosity of 3D biomaterial scaffolds and osteogenesis. Biomaterials, 26(27): 5474–5491. https://doi.org/10.1016/j.biomaterials.2005.02.002
9. Kuboki Y, Jin Q, Takita H, 2001, Geometry of carriers controlling phenotypic expression in BMP-induced osteogenesis and chondrogenesis. J Bone Joint Surg (Am Vol), 83-A Suppl 1(Pt 2): S105–S115.
10. Kuboki Y, Takita H, Kobayashi D, et al., 1998, BMP-induced osteogenesis on the surface of hydroxyapatite with geometrically feasible and nonfeasible structures: Topology of osteogenesis. J Biomed Mater Res, 39(2): 190–199.
11. Tsuruga E, Takita H, Itoh H, et al., 1997, Pore size of porous hydroxyapatite as the cell-substratum controls BMP-induced osteogenesis. J Biochem, 121(2): 317–324.
12. von Doernberg M-C, von Rechenberg B, Bohner M, et al., 2006, In vivo behavior of calcium phosphate scaffolds with four different pore sizes. Biomaterials, 27(30): 5186–5198. http://dx.doi.org/10.1016/j.biomaterials.2006.05.051
13. Ghayor C, Weber FE, 2018, Osteoconductive microarchitecture of bone substitutes for bone regeneration revisited. Front Physiol, 9: 960. https://doi.org/10.3389/fphys.2018.00960
14. Cornell CN, Lane JM, 1998, Current understanding of osteoconduction in bone regeneration. Clin Orthop Relat Res, 355: S267–S273.
15. Urist MR, 1976, Practical applications of basic research on bone graft physiology. The American Academy of Orthopaedic Surgeons: Instructional Course Lectures, Vol XXV, The C.V. Mosby Company, St Louis, 1–26.
16. Li J, Liu Y, Zhang Y, et al., 2021, Biophysical and biochemical cues of biomaterials guide mesenchymal stem cell behaviors. Front Cell Dev Biol, 9: 640388. https://doi.org/10.3389/fcell.2021.640388
17. Horwitz R, Webb D, 2003, Cell migration. Curr Biol, 13(19): R756–R759. https://doi.org/10.1016/j.cub.2003.09.014
18. Mayor R, Etienne-Manneville S, 2016, The front and rear of collective cell migration. Nat Rev Mol Cell Biol, 17(2): 97–109. https://doi.org/10.1038/nrm.2015.14
19. Schwartz MA, Horwitz AR, 2006, Integrating adhesion, protrusion, and contraction during cell migration. Cell, 125(7): 1223–1225. https://doi.org/10.1016/j.cell.2006.06.015
20. Qian T, Wang Y, 2010, Micro/nano-fabrication technologies for cell biology. Med Biol Eng Comput, 48(10): 1023–1032. https://doi.org/10.1007/s11517-010-0632-z
21. Diloksumpan P, Bolaños RV, Cokelaere S, et al., 2020, Orthotopic bone regeneration within 3D printed bioceramic scaffolds with region-dependent porosity gradients in an equine model. Adv Healthc Mater, 9(10): 1901807. https://doi.org/10.1002/adhm.201901807
22. Xue J, Wu T, Xia Y, 2018, Perspective: Aligned arrays of electrospun nanofibers for directing cell migration. APL Mater, 6(12): 120902. https://doi.org/10.1063/1.5058083
23. Berner A, Woodruff MA, Lam CX, et al., 2014, Effects of scaffold architecture on cranial bone healing. Int J Oral Maxillofac Surg, 43(4): 506–513. https://doi.org/10.1016/j.ijom.2013.05.008
24. Crump SS, 1992, Apparatus und method for creating tree-dimensional objects. Patent, No. 5121329.
25. Millán AJ, Santacruz I, Sánchez-Herencia AJ, et al., 2002, Gel-extrusion: A new continuous forming technique. Adv Eng Mater, 4(12): 913–915. https://doi.org/10.1002/adem.200290002
26. Vozzi G, Previti A, De Rossi D, et al., 2002, Microsyringe-based deposition of two-dimensional and three-dimensional polymer scaffolds with a well-defined geometry for application to tissue engineering. Tissue Eng, 8(6): 1089–1098. https://doi.org/10.1089/107632702320934182
27. Hutmacher DW, Schantz T, Zein I, et al., 2001, Mechanical properties and cell cultural response of polycaprolactone scaffolds designed and fabricated via fused deposition modeling. J Biomed Mater Res, 55: 203–216.
28. Schantz JT, Lim TC, Ning C, et al., 2006, Cranioplasty after trephination using a novel biodegradable burr hole cover: Technical case report. Neurosurgery, 58(1 Suppl): ONS-E176; discussion ONS-E176. https://doi.org/10.1227/01.NEU.0000193533.54580.3F
29. Lin C, Wang Y, Huang Z, et al., 2021, Advances in filament structure of 3D bioprinted biodegradable bone repair scaffolds. Int J Bioprint [Internet], 7(4): 426. https://doi.org/10.18063/ijb.v7i4.426
30. Ghayor C, Chen TH, Bhattacharya I, et al., 2020, Microporosities in 3D-printed tricalcium-phosphate-based bone substitutes enhance osteoconduction and affect osteoclastic resorption. Int J Mol Sci, 21(23): 9270. https://doi.org/10.3390/ijms21239270
31. de Wild M, Zimmermann S, Ruegg J, et al., 2016, Influence of microarchitecture on osteoconduction and mechanics of porous titanium scaffolds generated by selective laser melting. 3d Print Addit Manuf, 3(3): 142–151. https://doi.org/10.1089/3dp.2016.0004
32. Chen TH, Ghayor C, Siegenthaler B, et al., 2018, Lattice microarchitecture for bone tissue engineering from calcium phosphate compared to titanium. Tissue Eng Part A, 24(19–20): 1554–1561. https://doi.org/10.1089/ten.TEA.2018.0014
33. Agrawal L, Saidani M, Guillaud L, et al., 2021, Development of 3D culture scaffolds for directional neuronal growth using 2-photon lithography. Mater Sci Eng C, 131: 112502. https://doi.org/10.1016/j.msec.2021.112502
34. Ghayor C, Bhattacharya I, Guerrero J, et al., 2022, 3D-printed HA-based scaffolds for bone regeneration: Microporosity, osteoconduction and osteoclastic resorption. Materials, 15(4): 1433.
35. Klenke FM, Liu Y, Yuan H, et al., 2008, Impact of pore size on the vascularization and osseointegration of ceramic bone substitutes in vivo. J Biomed Mater Res A, 85(3): 777–786. https://doi.org/10.1002/jbm.a.31559
36. Mastrullo V, Cathery W, Velliou E, et al., 2020, Angiogenesis in tissue engineering: As nature intended? Front Bioeng Biotechnol, 8: 188. https://doi.org/10.3389/fbioe.2020.00188
37. Yang G, Mahadik B, Choi JY, et al., 2020, Vascularization in tissue engineering: Fundamentals and state-of-art. Prog Biomed Eng (Bristol), 2(1): 012002. https://doi.org/10.1088/2516-1091/ab5637
38. Wu F, Yang J, Ke X, et al., 2022, Integrating pore architectures to evaluate vascularization efficacy in silicate-based bioceramic scaffolds. Regen Biomater [Internet]. 9: rbab077. https://doi.org/10.1093/rb/rbab077
39. de Wild M, Ghayor C, Zimmermann S, et al., 2019, Osteoconductive lattice microarchitecture for optimized bone regeneration. 3D Print Addit Manuf, 6(1): 40–49. https://doi.org/10.1089/3dp.2017.0129
40. Huber F, Vollmer D, Vinke J, et al., 2022, Influence of 3D printing parameters on the mechanical stability of PCL scaffolds and the proliferation behavior of bone cells. Materials (Basel, Switzerland) [Internet]. 15(6): 2091. https://doi.org/10.3390/ma15062091