Thermo-sensitive Sacrificial Microsphere-based Bioink for Centimeter-scale Tissue with Angiogenesis
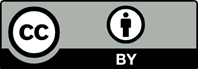
Centimeter-scale tissue with angiogenesis has become more and more significant in organ regeneration and drug screening. However, traditional bioink has obvious limitations such as balance of nutrient supporting, printability, and vascularization. Here, with “secondary bioprinting” of printed microspheres, an innovative bioink system was proposed, in which the thermo-crosslinked sacrificial gelatin microspheres encapsulating human umbilical vein endothelial cells (HUVECs) printed by electrospraying serve as auxiliary component while gelatin methacryloyl precursor solution mixed with subject cells serve as subject component. Benefiting from the reversible thermo-crosslinking feature, gelatin microspheres would experience solid-liquid conversion during 37°C culturing and form controllable porous nutrient network for promoting the nutrient/oxygen delivery in large-scale tissue and accelerate the functionalization of the encapsulated cells. Meanwhile, the encapsulated HUVECs would be released and attach to the pore boundary, which would further form three-dimensional vessel network inside the tissue with suitable inducing conditions. As an example, vascularized breast tumor tissue over 1 cm was successfully built and the HUVECs showed obvious sprout inside, which indicate the great potential of this bioink system in various biomedical applications.
1. He Y, Gu Z, Xie M, et al., 2020, Why Choose 3D Bioprinting? Part II: Methods and Bioprinters. BioDesign Manuf, 3:1–4. https://doi.org/10.1007/s42242-020-00064-w
2. Thakor J, Ahadian S, Niakan A, et al., 2020, Engineered Hydrogels for Brain Tumor Culture and Therapy. BioDesign Manuf, 3:203–26. https://doi.org/10.1007/s42242-020-00084-6
3. Lee M, Bae K, Guillon P, et al., 2018, Exploitation of Cationic Silica Nanoparticles for Bioprinting of Large-Scale Constructs with High Printing Fidelity. ACS Appl Mater Interfaces, 10:37820–8. https://doi.org/10.1021/acsami.8b13166
4. Ying GL, Jiang N, Maharjan S, et al., 2018, Aqueous Two-Phase Emulsion Bioink-Enabled 3D Bioprinting of Porous Hydrogels. Adv Mater, 30:1805460. https://doi.org/10.1002/adma.201805460
5. Shao L, Gao Q, Xie C, et al., 2020, Sacrificial Microgel-laden Bioink-enabled 3D Bioprinting of Mesoscale Pore Networks. BioDesign Manuf, 3:30–9. https://doi.org/10.1007/s42242-020-00062-y
6. Eilken HM, Adams RH, 2010, Dynamics of Endothelial Cell Behavior in Sprouting Angiogenesis. Curr Opin Cell Biol, 22:617–25. https://doi.org/10.1016/j.ceb.2010.08.010
7. Eng G, Lee BW, Parsa H, et al., 2013, Assembly of Complex Cell Microenvironments using Geometrically Docked Hydrogel Shapes. Proc Natl Acad Sci, 110:4551–6. https://doi.org/10.1073/pnas.1300569110
8. Lee VK, Kim DY, Ngo H, et al., 2014, Creating Perfused Functional Vascular Channels using 3D Bio-printing Technology. Biomaterials, 35:8092–102. https://doi.org/10.1016/j.biomaterials.2014.05.083
9. Nakatsu MN, Sainson RC, Aoto JN, et al., 2003, Angiogenic Sprouting and Capillary Lumen Formation Modeled by Human Umbilical Vein Endothelial Cells (HUVEC) in Fibrin Gels: The Role of Fibroblasts and Angiopoietin-1. Microvasc Res, 66:102–12. https://doi.org/10.1016/s0026-2862(03)00045-1
10. Kratochvil MJ, Seymour AJ, Li TL, et al., 2019, Engineered Materials for Organoid Systems. Nat Rev Mater, 4:606–22. https://doi.org/10.1038/s41578-019-0129-9
11. Daly AC, Riley L, Segura T, et al., 2019, Hydrogel Microparticles for Biomedical Applications. Nat Rev Mater, 5:20–43. https://doi.org/10.1038/s41578-019-0148-6
12. Parsa S, Gupta M, Loizeau F, et al., 2010, Effects of Surfactant and Gentle Agitation on Inkjet Dispensing of Living Cells. Biofabrication, 2:025003. https://doi.org/10.1088/1758-5082/2/2/025003
13. Caldwell AS, Campbell GT, Shekiro KM, et al., 2017, Clickable Microgel Scaffolds as Platforms for 3D Cell Encapsulation. Adv Healthc Mater, 6:254. https://doi.org/10.1002/adhm.201770080
14. Leong W, Lau TT, Wang DA, 2013, A Temperature-cured Dissolvable Gelatin Microsphere-based Cell Carrier for Chondrocyte Delivery in a Hydrogel Scaffolding System. Acta Biomater, 9:6459–67. https://doi.org/10.1016/j.actbio.2012.10.047
15. Chen X, Bai S, Li B, et al., 2016, Fabrication of Gelatin Methacrylate/Nanohydroxyapatite Microgel Arrays for Periodontal Tissue Regeneration. Int J Nanomed, 11:4707–18. https://doi.org/10.2147/ijn.s111701
16. Sinclair A, O’Kelly MB, Bai T, et al., 2018, Self-healing Zwitterionic Microgels as a Versatile Platform for Malleable Cell Constructs and Injectable Therapies. Adv Mater, 30:e1803087. https://doi.org/10.1002/adma.201870291
17. Seeto WJ, Tian Y, Winter RL, et al., 2017, Encapsulation of Equine Endothelial Colony Forming Cells in Highly Uniform, Injectable Hydrogel Microspheres for Local Cell Delivery. Tissue Eng Part C Methods, 23:815–25. https://doi.org/10.1089/ten.tec.2017.0233
18. Xie M, Gao Q, Fu J, et al., 2020, Bioprinting of Novel 3D Tumor Array Chip for Drug Screening. BioDesign Manuf, 3:175–88. https://doi.org/10.1007/s42242-020-00078-4
19. Cai S, Shi H, Li G, et al., 2019, 3D-printed Concentration controlled Microfluidic Chip with Diffusion Mixing Pattern for the Synthesis of Alginate Drug Delivery Microgels. Nanomaterials (Basel), 9:1451. https://doi.org/10.3390/nano9101451
20. Highley CB, Song KH, Daly AC, et al., 2019, Jammed Microgel Inks for 3D Printing Applications. Adv Sci (Weinh), 6:1801076. https://doi.org/10.1002/advs.201801076
21. An C, Liu W, Zhang Y, et al., 2020, Continuous Microfluidic Encapsulation of Single Mesenchymal Stem Cells using Alginate Microgels as Injectable Fillers for Bone Regeneration. Acta Biomater, 111:181–96. https://doi.org/10.1016/j.actbio.2020.05.024
22. Hinton TJ, Jallerat Q, Palchesko RN, et al., 2015, Three-dimensional Printing of Complex Biological Structures by Freeform Reversible Embedding of Suspended Hydrogels. Sci Adv, 1:e1500758. https://doi.org/10.1126/sciadv.1500758
23. Jeon O, Lee YB, Jeong H, et al., 2019, Individual Cellonly Bioink and Photocurable Supporting Medium for 3D Printing and Generation of Engineered Tissues with Complex Geometries. Mater Horiz, 6:1625–31. https://doi.org/10.1039/c9mh00375d
24. Khan MR, Sadiq MB, 2020, Importance of Gelatin, Nanoparticles and their Interactions in the Formulation of Biodegradable Composite Films: A Review. Polym Bull, 78:4047-73. https://doi.org/10.1007/s00289-020-03283-4
25. Ranasinghe RA, Wijesekara WL, Perera PR, et al., 2022, Functional and Bioactive Properties of Gelatin Extracted from Aquatic Bioresources-a Review. Food Rev Int, 38,4: 812-55. https://doi.org/10.1080/87559129.2020.1747486
26. Rigueto CV, Nazari MT, Massuda LÁ, et al., 2021, Production and Environmental Applications of Gelatin-based Composite Adsorbents for Contaminants Removal: A Review. Environ Chem Lett, 18:3. https://doi.org/10.1007/s10311-021-01184-0
27. Rodríguez-Rodríguez R, Espinosa-Andrews H, Velasquillo-Martínez C, et al., 2020, Composite Hydrogels Based on Gelatin, Chitosan and Polyvinyl Alcohol to Biomedical Applications: A Review. Int J Polym Mater Polym Biomater, 69:1–20. https://doi.org/10.1080/00914037.2019.1581780
28. Yang Z, Chaieb S, Hemar Y, 2021, Gelatin-based Nanocomposites: A Review. Polym Rev, 61:1–49. https://doi.org/10.1080/15583724.2021.1897995
29. Shoulders MD, Raines RT, 2009, Collagen Structure and Stability. Ann Rev Biochem, 78:929–58. https://doi.org/10.1146/annurev.biochem.77.032207.120833
30. Marini JC, Forlino A, Cabral WA, et al., 2007, Consortium for Osteogenesis Imperfecta Mutations in the Helical Domain of Type I Collagen: Regions Rich in Lethal Mutations Align with Collagen Binding Sites for Integrins and Proteoglycans. Hum Mutation, 28:209–21. https://doi.org/10.1002/humu.20429
31. Xie M, Gao Q, Zhao H, et al., 2019, Electro-assisted Bioprinting of Low-concentration GelMA Microdroplets. Small, 15:1804216. https://doi.org/10.1002/smll.201804216
32. Janmaleki M, Liu J, Kamkar M, et al., 2020, Role of Temperature on Bio-printability of Gelatin Methacryloyl Bioink in Two-step Cross-linking Strategy for Tissue Engineering Applications. Biomed Mater, 16:015021. https://doi.org/10.1088/1748-605x/abbcc9
33. Lee JY, Kim GH, 2020, A Cryopreservable Cell-laden GelMa-based Scaffold Fabricated using a 3D Printing Process Supplemented with an In Situ Photo-crosslinking. J Indust Eng Chem, 85:249–57. https://doi.org/10.1016/j.jiec.2020.02.007
34. Li H, Tan YJ, Kiran R, et al., 2021, Submerged and Non submerged 3D Bioprinting Approaches for the Fabrication of Complex Structures with the Hydrogel Pair GelMA and Alginate/Methylcellulose. Addit Manuf, 37:101640. https://doi.org/10.1016/j.addma.2020.101640
35. Rastin H, Ormsby RT, Atkins GJ, et al., 2020, 3D Bioprinting of Methylcellulose/Gelatin-methacryloyl (MC/GelMA) Bioink with High Shape Integrity. ACS Appl Bio Mater, 3:1815–26. https://doi.org/10.1021/acsabm.0c00169
36. Wang Y, Kankala RK, Zhu K, et al., 2019, Coaxial Extrusion of Tubular Tissue Constructs using a Gelatin/GelMA Blend Bioink. ACS Biomater Sci Eng, 5:5514–24. https://doi.org/10.1021/acsbiomaterials.9b00926
37. Albig AR, Schiemann WP, 2004, Fibulin-5 Antagonizes Vascular Endothelial Growth Factor (VEGF) Signaling and Angiogenic Sprouting by Endothelial Cells. DNA Cell Biol, 23:367–79. https://doi.org/10.1089/104454904323145254
38. Gerhardt H, Golding M, Fruttiger M, et al., 2003, VEGF Guides Angiogenic Sprouting Utilizing Endothelial Tip Cell Filopodia. J Cell Biol, 161:1163–77. https://doi.org/10.1083/jcb.200302047
39. Grashoff C, Hoffman BD, Brenner MD, et al., 2010, Measuring Mechanical Tension Across Vinculin Reveals Regulation of Focal Adhesion Dynamics. Nature, 466:263–6. https://doi.org/10.1038/nature09198
40. Humphries JD, Wang P, Streuli C, et al., 2007, Vinculin Controls Focal Adhesion Formation by Direct Interactions with Talin and Actin. J Cell Biol, 179:1043–57. https://doi.org/10.1083/jcb.200703036
41. Ziegler WH, Liddington RC, Critchley DR, 2006, The Structure and Regulation of Vinculin. Trends Cell Biol, 16:453–60. https://doi.org/10.1016/j.tcb.2006.07.004
42. Dejana E, Bazzoni G, Lampugnani MG, 1999, Vascular Endothelial (VE)-cadherin: Only an Intercellular Glue? Exp Cell Res, 252:13–9. https://doi.org/10.1006/excr.1999.4601
43. Dejana E, Orsenigo F, Lampugnani MG, 2008, The Role of Adherens Junctions and VE-cadherin in the Control of Vascular Permeability. J Cell Sci, 121:2115–22. https://doi.org/10.1242/jcs.017897
44. Vestweber D, 2008, VE-cadherin: The Major Endothelial Adhesion Molecule Controlling Cellular Junctions and Blood Vessel Formation. Arterioscler Thromb Vasc Biol, 28:223–32. https://doi.org/10.1161/atvbaha.107.158014