Enhanced Attachment and Collagen Type I Deposition of MC3T3-E1 Cells via Electrohydrodynamic Printed Sub-Microscale Fibrous Architectures
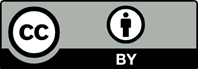
Micro/sub-microscale fibrillar architectures of extracellular matrix play important roles in regulating cellular behaviors such as attachment, migration, and differentiation. However, the interactions between cells and organized micro/ sub-microscale fibers have not been fully clarified yet. Here, the responses of MC3T3-E1 cells to electrohydrodynamic (EHD) printed scaffolds with microscale and/or sub-microscale fibrillar architectures were investigated to demonstrate their potential for bone tissue regeneration. Fibrillar scaffolds were EHD-fabricated with microscale (20.51 ± 1.70 μm) and/or sub-microscale (0.58 ± 0.51 μm) fibers in a controlled manner. The in vitro results showed that cells exhibited a 1.25-fold increase in initial attached cell number and 1.17-fold increase in vinculin expression on scaffolds with micro/sub-microscale fibers than that on scaffolds with pure microscale fibers. After 14 days of culture, the cells expressed 1.23 folds increase in collagen type I (COL-I) deposition compared with that on scaffolds with pure microscale fibers. These findings indicated that the EHD printed sub-microscale fibrous architectures can facilitate attachment and COL I secretion of MC3T3-E1 cells, which may provide a new insight to the design and fabrication of fibrous scaffolds for bone tissue engineering.
1. Eliaz Nand Metoki N, 2017, Calcium Phosphate Bioceramics: A Review of Their History, Structure, Properties, Coating Technologies and Biomedical Applications. Materials (Basel), 10:334. https://doi.org/10.3390/ma10040334
2. Florencio-Silva R, Sasso G R, Sasso-Cerri E, et al., 2015, Biology of Bone Tissue: Structure, Function, and Factors That Influence Bone Cells. Biomed Res Int, 2015:421746. https://doi.org/10.1155/2015/421746
3. Lin X, Patil S, Gao Y G, et al., 2020, The Bone Extracellular Matrix in Bone Formation and Regeneration. Front Pharmacol, 11:757. https://doi.org/10.3389/fphar.2020.00757
4. Hogrebe NJ, Reinhardt JW, Gooch KJ, 2017, Biomaterial Microarchitecture: A Potent Regulator of Individual Cell Behavior and Multicellular Organization. J Biomed MATER Res A, 105:640–61. https://doi.org/10.1002/jbm.a.35914
5. Xue J, Wu T, Dai Y, et al., 2019, Electrospinning and Electrospun Nanofibers: Methods, Materials, and Applications. Chem Rev, 119:5298–415. https://doi.org/10.1021/acs.chemrev.8b00593
6. Nekounam H, Samadian H, Bonakdar S, et al., 2021, Electro-Conductive Carbon Nanofibers Containing Ferrous Sulfate for Bone Tissue Engineering. Life Sci, 282:119602. https://doi.org/10.1016/j.lfs.2021.119602
7. Wu L, Gu Y, Liu L, et al., 2020, Hierarchical Micro/Nanofibrous Membranes of Sustained Releasing VEGF for Periosteal Regeneration. Biomaterials, 227:119555. https://doi.org/10.1016/j.biomaterials.2019.119555
8. Yao Q, Cosme J G, Xu T, et al., 2017, Three Dimensional Electrospun PCL/PLA Blend Nanofibrous Scaffolds with Significantly Improved Stem Cells Osteogenic Differentiation and Cranial Bone Formation. Biomaterials, 115:115–27. https://doi.org/10.1016/j.biomaterials.2016.11.018
9. Ren K, Wang Y, Sun T, et al., 2017, Electrospun PCL/Gelatin Composite Nanofiber Structures for Effective Guided Bone Regeneration Membranes. Mater Sci Eng C Mater Biol Appl, 78:324–32. https://doi.org/10.1016/j.msec.2017.04.084
10. Bean AC, Tuan RS, 2015, Fiber Diameter and Seeding Density Influence Chondrogenic Differentiation of Mesenchymal Stem Cells Seeded on Electrospun Poly (Epsilon-Caprolactone) Scaffolds. Biomed Mater, 10:015018. https://doi.org/10.1088/1748-6041/10/1/015018
11. Shanmugasundaram S, Chaudhry H, Arinzeh TL, 2011, Microscale Versus Nanoscale Scaffold Architecture for Mesenchymal Stem Cell Chondrogenesis. Tissue Eng Part A, 17:831–40. https://doi.org/10.1089/ten.TEA.2010.0409
12. Sisson K, Zhang C, Farach-Carson MC, et al., 2010, Fiber Diameters Control Osteoblastic Cell Migration and Differentiation in Electrospun Gelatin. J Biomed Mater Res A, 94:1312–20. https://doi.org/10.1002/jbm.a.32756
13. He J, Zhang B, Li Z, et al., 2020, High-Resolution Electrohydrodynamic Bioprinting: A New Biofabrication Strategy for Biomimetic Micro/Nanoscale Architectures and Living Tissue Constructs. Biofabrication, 12:042002. https://doi.org/10.1088/1758-5090/aba1fa
14. Abbasi N, Abdal-hay A, Hamlet S, et al., 2019, Effects of Gradient and Offset Architectures on the Mechanical and Biological Properties of 3-D Melt Electrowritten (MEW) Scaffolds. ACS Biomater Sci Eng, 5:3448–61. https://doi.org/10.1021/acsbiomaterials.8b01456
15. Abbasi N, Ivanovski S, Gulati K, et al., 2020, Role of Offset and Gradient Architectures of 3-D Melt Electrowritten Scaffold on Differentiation and Mineralization of Osteoblasts. Biomater Res, 24:2. https://doi.org/10.1186/s40824-019-0180-z
16. Mao M, He J, Li Z, et al., 2020, Multi-Directional Cellular Alignment in 3D Guided by Electrohydrodynamically-Printed Microlattices. Acta Biomater, 101:141–51. https://doi.org/10.1016/j.actbio.2019.10.028
17. Brennan CM, Eichholz KF, Hoey DA, 2019, The Effect of Pore Size within Fibrous Scaffolds Fabricated Using Melt Electrowriting on Human Bone Marrow Stem Cell Osteogenesis. Biomed Mater, 14:065016. https://doi.org/10.1088/1748-605X/ab49f2
18. Eichholz KF, Hoey DA, 2018, Mediating Human Stem Cell Behaviour Via Defined Fibrous Architectures by Melt Electrospinning Writing. Acta Biomater, 75:140–51. https://doi.org/10.1016/j.actbio.2018.05.048
19. Xie C, Gao Q, Wang P, et al., 2019, Structure-Induced Cell Growth by 3D Printing of Heterogeneous Scaffolds with Ultrafine Fibers. Mater Des, 181:108092. https://doi.org/10.1016/j.matdes.2019.108092
20. Zhang B, He J, Lei Q, et al., 2019, Electrohydrodynamic Printing of Sub-Microscale Fibrous Architectures with Improved Cell Adhesion Capacity. Virtual Phys Prototyp, 15:62-74. https://doi.org/10.1080/17452759.2019.1662991
21. He J, Xu F, Dong R, et al., 2017, Electrohydrodynamic 3D Printing of Microscale Poly (Epsilon-Caprolactone) Scaffolds with Multi-Walled Carbon Nanotubes. Biofabrication, 9:015007. https://doi.org/10.1088/1758-5090/aa53bc
22. Vijayavenkataraman S, Thaharah S, Zhang S, et al., 2019, 3D-Printed PCL/rGO Conductive Scaffolds for Peripheral Nerve Injury Repair. Artif Organs, 43:515–23. https://doi.org/10.1111/aor.13360
23. Zhou Hand Lee J, 2011, Nanoscale Hydroxyapatite Particles for Bone Tissue Engineering. Acta Biomater, 7:2769–81. https://doi.org/10.1016/j.actbio.2011.03.019
24. Turnbull G, Clarke J, Picard F, et al., 2018, 3D Bioactive Composite Scaffolds for Bone Tissue Engineering. Bioact Mater, 3:278–314. https://doi.org/10.1016/j.bioactmat.2017.10.001
25. Wu X, Miao L, Yao Y, et al., 2014, Electrospun Fibrous Scaffolds Combined with Nanoscale Hydroxyapatite Induce Osteogenic Differentiation of Human Periodontal Ligament Cells. Int J Nanomed, 9:4135–43. https://doi.org/10.2147/IJN.S65272
26. Ribeiro Neto WA, Pereira IH, Ayres E, et al., 2012, Influence of the Microstructure and Mechanical Strength of Nanofibers of Biodegradable Polymers with Hydroxyapatite in Stem Cells Growth. Electrospinning, Characterization and Cell Viability. Polym Degrad Stab, 97:2037–51. https://doi.org/10.1016/j.polymdegradstab.2012.03.048
27. Li B, Chen Y, He J, et al., 2020, Biomimetic Membranes of Methacrylated Gelatin/Nanohydroxyapatite/Poly(l-Lactic Acid) for Enhanced Bone Regeneration. ACS Biomater Sci Eng, 6:6737–47. https://doi.org/10.1021/acsbiomaterials.0c00972
28. Lei Q, He J, Li D, 2019, Electrohydrodynamic 3D Printing of Layer-Specifically Oriented, Multiscale Conductive Scaffolds for Cardiac Tissue Engineering. Nanoscale, 11:15195–205. https://doi.org/10.1039/c9nr04989d
29. Xia J, Yuan Y, Wu H, et al., 2020, Decoupling the Effects of Nanopore Size and Surface Roughness on the Attachment, Spreading and Differentiation of Bone Marrow-Derived Stem Cells. Biomaterials, 248:120014. https://doi.org/10.1016/j.biomaterials.2020.120014
30. Gao C, Peng S, Feng P, et al., 2017, Bone Biomaterials and Interactions with Stem Cells. Bone Res, 5:17059. https://doi.org/10.1038/boneres.2017.59
31. Tian F, Hosseinkhani H, Hosseinkhani M, et al., 2008, Quantitative Analysis of Cell Adhesion on Aligned Microand Nanofibers. J Biomed Mater Res A, 84:291–9. https://doi.org/10.1002/jbm.a.31304
32. Huang B, Aslan E, Jiang Z, et al., 2020, Engineered Dual-Scale Poly (ε-Caprolactone) Scaffolds using 3D Printing and Rotational Electrospinning for Bone Tissue Regeneration. Addit Manuf, 36:101452. https://doi.org/10.1016/j.addma.2020.101452
33. Oakes PW, Gardel ML, 2014, Stressing the Limits of Focal Adhesion Mechanosensitivity. Curr Opin Cell Biol, 30:68–73. https://doi.org/10.1016/j.ceb.2014.06.003
34. Paluch EK, Aspalter IM, Sixt M, 2016, Focal Adhesion-Independent Cell Migration. Annu Rev Cell Dev Biol, 32:469–90. https://doi.org/10.1146/annurev-cellbio-111315-125341
35. Revach OY, Grosheva I, Geiger B, 2020, Biomechanical Regulation of Focal Adhesion and Invadopodia Formation. J Cell Sci, 133:jcs244848. https://doi.org/10.1242/jcs.244848
36. Sheets K, Wunsch S, Ng C, et al., 2013, Shape-Dependent Cell Migration and Focal Adhesion Organization on Suspended and Aligned Nanofiber Scaffolds. Acta Biomater, 9:7169–77. https://doi.org/10.1016/j.actbio.2013.03.042
37. Fu X, Liu G, Halim A, et al., 2019, Mesenchymal Stem Cell Migration and Tissue Repair. Cells, 8:784. https://doi.org/10.3390/cells8080784
38. Abedin E, Lari R, Shahri NM, et al., 2018, Development of a Demineralized and Decellularized Human Epiphyseal Bone Scaffold for Tissue Engineering: A Histological Study. Tissue Cell, 55:46–52. https://doi.org/10.1016/j.tice.2018.09.003
39. Blair HC, Larrouture QC, Li Y, et al., 2017, Osteoblast Differentiation and Bone Matrix Formation In Vivo and In Vitro. Tissue Eng Part B Rev, 23:268–80. https://doi.org/10.1089/ten.TEB.2016.0454
40. Oryan A, Kamali A, Moshiri A, et al., 2017, Role of Mesenchymal Stem Cells in Bone Regenerative Medicine: What Is the Evidence? Cells Tissues Organs, 204:59–83. https://doi.org/10.1159/000469704
41. Iaquinta MR, Mazzoni E, Bononi I, et al., 2019, Adult Stem Cells for Bone Regeneration and Repair. Front Cell Dev Biol, 7:268. https://doi.org/10.3389/fcell.2019.00268