Nano-Hydroxyapatite Bone Scaffolds with Different Porous Structures Processed by Digital Light Processing 3D Printing
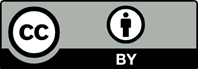
The morphologies and structures of the scaffold have a significant influence on their mechanical and biological properties. In this work, different types of porous structures: Triply periodic minimal surface-Schwarz primitive (P), bodycentered cubic, and cubic pore-shaped (CPS) hydroxyapatite scaffolds with ~70% porosity were fabricated through digital light processing (DLP) 3D printing technology. The compressive properties and in vitro cell evaluations such as cell proliferation and attachment morphology of these scaffolds were systematically compared. The results showed that the CPS scaffolds exhibited the highest compressive strength (~22.5 MPa) and modulus (~400 MPa). In addition, the CPS scaffolds also performed the most active cell metabolisms as compared to other two structures, which may account for the larger pore size and smaller curvature of the substrate. This study provides a general guidance for the fabrication and selection of porous bone scaffolds processed by DLP 3D printing.
1. Pilia M, Guda T, Appleford M, 2013, Development of Composite Scaffolds for Load-Bearing Segmental Bone Defects. Biomed Res Int, 2013:458253. https://doi.org/10.1155/2013/458253
2. Wang X, Ao Q, Tian X, et al., 2016, 3D Bioprinting Technologies for Hard Tissue and Organ Engineering. Materials, 9:802. https://doi.org/10.3390/ma9100802
3. Lin K, Sheikh R, Romanazzo S, et al., 2019, 3D Printing of bioceramic Scaffolds-Barriers to the Clinical Translation: From Promise to Reality, and Future Perspectives. Materials, 12:2660. https://doi.org/10.3390/ma12172660
4. Goodman SB, Maruyama M, 2020, Inflammation, Bone Healing and Osteonecrosis: From Bedside to Bench. J Inflamm Res, 13:913. https://doi.org/10.2147/jir.s281941
5. Keating JF, Simpson A, Robinson C, 2005, The Management of Fractures with Bone Loss. J Bone Joint Surg Br, 87:142–50. https://doi.org/10.1302/0301-620X.87B2.15874
6. Sanders DW, Bhandari M, Guyatt G, et al., 2014, Critical-Sized Defect in the Tibia: Is It Critical? Results from the SPRINT Trial. J Orthop Trauma, 28:632–5. https://doi.org/10.1097/BOT.0000000000000194
7. McDermott AM, Herberg S, Mason DE, et al., 2019, Recapitulating Bone Development Through Engineered Mesenchymal Condensations and Mechanical Cues for Tissue Regeneration. Sci Transl Med, 11:eaav7756. https://doi.org/10.1126/scitranslmed.aav7756
8. Zhu Y, Zhang K, Zhao R, et al., 2017, Bone Regeneration with Micro/Nano Hybrid-Structured Biphasic Calcium Phosphate Bioceramics at Segmental Bone Defect and the Induced Immunoregulation of MSCs. Biomaterials, 147:133–44. https://doi.org/10.1016/j.biomaterials.2017.09.018
9. Klar RM, 2018, The Induction of Bone Formation: The Translation Enigma. Front Bioeng Biotechnol, 6:74. https://doi.org/10.3389/fbioe.2018.00074
10. Roddy E, DeBaun MR, Daoud-Gray A, et al., 2018, Treatment of Critical-Sized Bone Defects: Clinical and Tissue Engineering Perspectives. Eur J Orthop Surg Traumatol, 28:351–62. https://doi.org/10.1007/s00590-017-2063-0
11. Khairallah M, Almeshaly H, 2016, Present Strategies for Critical Bone Defects Regeneration. Oral Health Case Rep, 2:3. https://doi.org/10.4172/2471-8726.1000127
12. Roseti L, Parisi V, Petretta M, et al., 2017, Scaffolds for Bone Tissue Engineering: State of the Art and New Perspectives. Mater Sci Eng C, 78:1246–62. https://doi.org/10.1016/j.msec.2017.05.017
13. Blanquer SB, Werner M, Hannula M, et al., 2017, Surface Curvature in Triply-Periodic Minimal Surface Architectures as a Distinct Design Parameter in Preparing Advanced Tissue Engineering Scaffolds. Biofabrication, 9:025001. https://doi.org/10.1088/1758-5090/aa6553
14. Lu F, Wu R, Shen M, et al., 2021, Rational Design of Bioceramic Scaffolds with Tuning Pore Geometry by Stereolithography: Microstructure Evaluation and Mechanical Evolution. J Eur Ceram Soc, 41:1672–82. https://doi.org/10.1016/j.jeurceramsoc.2020.10.002
15. Yao Y, Qin W, Xing B, et al., 2021, High Performance Hydroxyapatite Ceramics and a Triply Periodic Minimum Surface Structure Fabricated by Digital Light Processing 3D Printing. J Adv Ceram, 10:39–48. https://doi.org/10.1007/s40145-020-0415-4
16. Huo P, Zhao Z, Bai P, et al., 2021, Deformation Evolution and Fracture Mechanism of Porous TC4 Alloy Scaffolds Fabricated Using Selective Laser Melting under Uniaxial Compression. J Alloys Compd, 861:158529. https://doi.org/10.1016/j.jallcom.2020.158529
17. Caravaggi P, Liverani E, Leardini A, et al., 2019, CoCr Porous Scaffolds Manufactured Via Selective Laser Melting in Orthopedics: Topographical, Mechanical, and Biological Characterization. J Biomed Mater Res B Appl Biomater, 107:2343–53. https://doi.org/10.1002/jbm.b.34328
18. Bigham A, Foroughi F, Ghomi ER, et al., 2020, The Journey of Multifunctional Bone Scaffolds Fabricated from Traditional Toward Modern Techniques. Biodes Manuf, 3:1–26. https://doi.org/10.1007/s42242-020-00094-4
19. Sun J, Binner J, Bai J, 2019, Effect of Surface Treatment on the Dispersion of Nano Zirconia Particles in Non-Aqueous Suspensions for Stereolithography. J Eur Ceram Soc, 39:1660–7. https://doi.org/10.1016/j.jeurceramsoc.2018.10.024
20. Ding G, He R, Zhang K, et al., 2020, Dispersion and Stability of SiC Ceramic Slurry for Stereolithography. Ceram Int, 46:4720–9. https://doi.org/10.1016/j.ceramint.2019.10.203
21. King BW Jr., 1940, Effect of Particle Size and Index of Refraction on Reflectance. J Am Ceram Soc, 23:221–5. https://doi.org/10.1111/j.1151-2916.1940.tb14258.x
22. Ju Y, Ha J, Song Y, et al., 2020, Optimizing the Printability and Dispersibility of Functionalized Zirconium Oxide/Acrylate Composites with Various Nano-to Micro-Particle Ratios. Ceram Int, 46:26903–10. https://doi.org/10.1016/j.ceramint.2020.07.168
23. De Camargo IL, Morais MM, Fortulan CA, et al., 2021, A Review on the Rheological Behavior and Formulations of Ceramic Suspensions for Vat Photopolymerization. Ceram Int, 47:11906–21. https://doi.org/10.1016/j.ceramint.2021.01.031
24. Sun J, Binner J, Bai J, 2020, 3D Printing of Zirconia Via Digital Light Processing: Optimization of Slurry and Debinding Process. J Eur Ceram Soc, 40:5837–44. https://doi.org/10.1016/j.jeurceramsoc.2020.05.079
25. Zheng T, Wang W, Sun J, et al., 2020, Development and Evaluation of Al2O3-ZrO2 Composite Processed by Digital Light 3D Printing. Ceram Int, 46:8682–8. https://doi.org/10.1016/j.ceramint.2019.12.102
26. Yu S, Sun J, Bai J, 2019, Investigation of Functionally Graded TPMS Structures Fabricated by Additive Manufacturing. Mater Des, 182:108021. https://doi.org/10.1016/j.matdes.2019.108021
27. Vijayavenkataraman S, Zhang L, Zhang S, et al., 2018, Triply Periodic Minimal Surfaces Sheet Scaffolds for Tissue Engineering Applications: An Optimization Approach Toward Biomimetic Scaffold Design. ACS Appl Bio Mater, 1:259–69. https://doi.org/10.1021/acsabm.8b00052
28. Cross MM, 1965, Rheology of non-Newtonian Fluids: A New Flow Equation for Pseudoplastic Systems. J Coll Sci, 20:417–37. https://doi.org/10.1016/0095-8522(65)90022-X
29. Barnes HA, Hutton JF, Walters K, 1989, An Introduction to Rheology. Amsterdam, Netherlands: Elsevier.
30. Griffith ML, Halloran JW, 1996, Freeform Fabrication of Ceramics Via Stereolithography. J Am Ceram Soc, 79:2601–8. https://doi.org/10.1111/j.1151-2916.1996.tb09022.x
31. Conti L, Bienenstein D, Borlaf M, et al., 2020, Effects of the Layer Height and Exposure Energy on the Lateral Resolution of Zirconia Parts Printed by Lithography-Based Additive Manufacturing. Materials, 13:1317. https://doi.org/10.3390/ma13061317
32. Wang K, Qiu M, Jiao C, et al., 2020, Study on Defect- Free Debinding Green Body of Ceramic Formed by DLP Technology. Ceram Int, 46:2438–46. https://doi.org/10.1016/j.ceramint.2019.09.237
33. Feng C, Zhang K, He R, et al., 2020, Additive Manufacturing of Hydroxyapatite Bioceramic Scaffolds: Dispersion, Digital Light Processing, Sintering, Mechanical Properties, and Biocompatibility. J Adv Ceram, 9:360–73. https://doi.org/10.1007/s40145-020-0375-8
34. Zeng Y, Yan Y, Yan H, et al., 2018, 3D Printing of Hydroxyapatite Scaffolds with Good Mechanical and Biocompatible Properties by Digital Light Processing. J Mater Sci, 53:6291–301. https://doi.org/10.1007/s10853-018-1992-2
35. Shan J, Yang Z, Chen G, et al., 2020, Design and Synthesis of Free-Radical/Cationic Photosensitive Resin Applied for 3D Printer with Liquid Crystal Display (LCD) Irradiation. Polymers, 12:1346. https://doi.org/10.3390/polym12061346
36. Guo B, Ji X, Wang W, et al., 2021, Highly Flexible, Thermally Stable, and Static Dissipative Nanocomposite with Reduced Functionalized Graphene Oxide Processed Through 3D Printing. Compos B Eng, 208:108598. https://doi.org/10.1016/j.compositesb.2020.108598
37. Karageorgiou V, Kaplan D, 2005, Porosity of 3D Biomaterial Scaffolds and Osteogenesis. Biomaterials, 26:5474–91. https://doi.org/10.1016/j.biomaterials.2005.02.002
38. Bružauskaitė I, Bironaitė D, Bagdonas E, et al., 2016, Scaffolds and Cells for Tissue Regeneration: Different Scaffold Pore Sizes-Different Cell Effects. Cytotechnology, 68:355–69. https://doi.org/10.1007/s10616-015-9895-4
39. Huri PY, Ozilgen BA, Hutton DL, et al., 2014, Scaffold Pore Size Modulates In Vitro Osteogenesis of Human Adipose-Derived Stem/Stromal Cells. Biomed Mater, 9:045003. https://doi.org/10.1088/1748-6041/9/4/045003
40. Velioglu ZB, Pulat D, Demirbakan B, et al., 2019, 3D-Printed Poly (Lactic Acid) Scaffolds for Trabecular Bone Repair and Regeneration: Scaffold and Native Bone Characterization. Connect Tissue Res, 60:274–82. https://doi.org/10.1080/03008207.2018.1499732
41. Edwards K, 2005, Selecting Materials for Optimum Use in Engineering Components. Mater Des, 26:469–73. https://doi.org/10.1016/j.matdes.2004.07.004
42. van Lenthe GH, de Waal Malefijt MC, Huiskes R, 1997, Stress Shielding after Total Knee Replacement May Cause Bone Resorption in the Distal Femur. J Bone Joint Surg Br, 79:117–22. https://doi.org/10.1302/0301-620X.79B1.0790117
43. Lindahl O, 1976, Mechanical Properties of Dried Defatted Spongy Bone. Acta Orthop Scand, 47:11–9. https://doi.org/10.3109/17453677608998966
44. Røhl L, Larsen E, Linde F, et al., 1991, Tensile and Compressive Properties of Cancellous Bone. J Biomech, 24:1143–9. https://doi.org/10.1016/0021-9290(91)90006-9
45. Giesen E, Ding M, Dalstra M, et al., 2001, Mechanical Properties of Cancellous Bone in the Human Mandibular Condyle are Anisotropic. J Biomech, 34:799–803. https://doi.org/10.1016/S0021-9290(01)00030-6
46. Xie J, Zorman J, Indrawati L, et al., 2013, Development and Optimization of a Novel Assay to Measure Neutralizing Antibodies Against Clostridium Difficile Toxins. Clin Vaccine Immunol, 20:517–25. https://doi/10.1128/CVI.00549-12
47. Lan Y, Jin Q, Xie H, et al., 2020, Exosomes Enhance Adhesion and Osteogenic Differentiation of Initial Bone Marrow Stem Cells on Titanium Surfaces. Front Cell Dev Biol, 8:583234. https://doi.org/10.3389/fcell.2020.583234
48. Zheng HZ, Fu XK, Shang JL, et al., 2018, Ginsenoside Rg1 Protects Rat Bone Marrow Mesenchymal Stem Cells Against Ischemia Induced Apoptosis Through miR-494-3p and ROCK-1. Eur J Pharmacol, 822:154–67. https://doi.org/10.1016/j.ejphar.2018.01.001
49. Sun Z, Yan K, Liu S, et al., 2021, Semaphorin 3A Promotes the Osteogenic Differentiation of Rat Bone Marrow-Derived Mesenchymal Stem Cells in Inflammatory Environments by Suppressing the Wnt/β-Catenin Signaling Pathway. J Mol Histol, 52:1245–125. https://doi.org/10.1007/s10735-020-09941-1
50. Lyu J, Hashimoto Y, Honda Y, et al., 2021, Comparison of Osteogenic Potentials of Dental Pulp and Bone Marrow Mesenchymal Stem Cells Using the New Cell Transplantation Platform, CellSaic, in a Rat Congenital Cleft-Jaw Model. Int J Mol Sci, 22:9478. https://doi.org/10.3390/ijms22179478
51. Li W, Liu Y, Wang B, et al., 2016, Protective Effect of Berberine Against Oxidative Stress-Induced Apoptosis in Rat Bone Marrow-Derived Mesenchymal Stem Cells. Exp Ther Med, 12:4041–8. https://doi.org/10.3892/etm.2016.3866
52. Sousa B, Pereira J, Paredes J, 2019, The Crosstalk Between Cell Adhesion and Cancer Metabolism. Int J Mol Sci, 20:1933. https://doi.org/10.3390/ijms20081933
53. Hamidi H, Ivaska J, 2021, Food for Thought: How Cell Adhesion Coordinates Nutrient Sensing. J Cell Biol, 220:e202103128. https://doi.org/10.1083/jcb.202103128
54. Ng IC, Pawijit P, Tan J, et al., 2019, Anatomy and Physiology for Biomaterials Research and Development. https://doi.org/10.1016/B978-0-12-801238-3.99876-3
55. Fritz V, Fajas L, 2010, Metabolism and Proliferation Share Common Regulatory Pathways in Cancer Cells. Oncogene, 29:4369–77. https://doi.org/10.1038/onc.2010.182
56. Chen QZ, Thouas GA, 2011, Fabrication and Characterization of Sol-Gel Derived 45S5 Bioglass®-Ceramic Scaffolds. Acta Biomater, 7:3616–26. https://doi.org/10.1016/j.actbio.2011.06.005
57. Díaz-Arca A, Ros-Tárraga P, Tomé MJ, et al., 2021, Micro-/Nano-Structured Ceramic Scaffolds That Mimic Natural Cancellous Bone. Materials, 14:1439. https://doi.org/10.3390/ma14061439
58. Liao B, Xia RF, Li W, et al., 2021, 3D-Printed Ti6Al4V Scaffolds with Graded Triply Periodic Minimal Surface Structure for Bone Tissue Engineering. J Mater Eng Perform, 30:4993–5004. https://doi.org/10.1007/s11665-021-05580-z
59. Perez RA, Mestres G, 2016, Role of Pore Size and Morphology in Musculo-Skeletal Tissue Regeneration. Mater Sci Eng C, 61:922–39. https://doi.org/10.1016/j.msec.2015.12.087
60. Lee SJ, Yang S, 2017, Substrate Curvature Restricts Spreading and Induces Differentiation of Human Mesenchymal Stem Cells. Biotechnol J, 12:1700360. https://doi.org/10.1002/biot.201700360
61. Ji L, LaPointe VL, Evans ND, et al., 2012, Changes in Embryonic Stem Cell Colony Morphology and Early Differentiation Markers Driven by Colloidal Crystal Topographical Cues. Eur Cell Mater, 23:135–46. https://doi.org/10.22203/eCM.v023a10
62. Chen W, Shao Y, Li X, et al., 2014, Nanotopographical Surfaces for Stem Cell Fate Control: Engineering Mechanobiology from the Bottom. Nano Today, 9:759–84. https://doi.org/10.1016/j.nantod.2014.12.002
63. Xia J, Yuan Y, Wu H, et al., 2020, Decoupling the Effects of Nanopore Size and Surface Roughness on the Attachment, Spreading and Differentiation of Bone Marrow-Derived Stem Cells. Biomaterials, 248:120014. https://doi.org/10.1016/j.biomaterials.2020.120014