Additive manufacturing of bone scaffolds
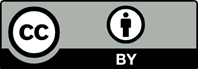
Additive manufacturing (AM) can obtain not only customized external shape but also porous internal structure for scaffolds, both of which are of great importance for repairing large segmental bone defects. The scaffold fabrication process generally involves scaffold design, AM, and post-treatments. Thus, this article firstly reviews the state-of-the-art of scaffold design, including computer-aided design, reverse modeling, topology optimization, and mathematical modeling. In addition, the current characteristics of several typical AM techniques, including selective laser sintering, fused deposition modeling (FDM), and electron beam melting (EBM), especially their advantages and limitations are presented. In particular, selective laser sintering is able to obtain scaffolds with nanoscale grains, due to its high heating rate and a short holding time. However, this character usually results in insufficient densification. FDM can fabricate scaffolds with a relative high accuracy of pore structure but with a relative low mechanical strength. EBM with a high beam-material coupling efficiency can process high melting point metals, but it exhibits a low-resolution and poor surface quality. Furthermore, the common post-treatments, with main focus on heat and surface treatments, which are applied to improve the comprehensive performance are also discussed. Finally, this review also discusses the future directions for AM scaffolds for bone tissue engineering.
1. Gao C, Peng S, Feng P, et al., 2017, Bone biomaterials and interactions with stem cells. Bone Res., 5(4): 17059.
2. Currey J D, 2012, The structure and mechanics of bone. J. Mater. Sci., 47(1): 41–54.
3. Heuijerjans A, Wilson W, Ito K, et al., 2017, The critical size of focal articular cartilage defects is associated with strains in the collagen fibers. Clin Biomech, 50: 40.
4. Yang Y, Wu P, Wang Q, et al., 2016, The enhancement of Mg corrosion resistance by alloying Mn and laser–melting. Materials, 9(4): 216.
5. Hoover S, Tarafder S, Bandyopadhyay A, et al., 2017, Silver doped resorbable tricalcium phosphate scaffolds for bone graft applications. Mater Sci Eng C Mater Biol Appl, 79: 763–769.
6. Faroni A, Mobasseri S A, Kingham P J, et al., 2015, Peripheral nerve regeneration: Experimental strategies and future perspectives. Adv Drug Deliv Rev, 82–83: 160–167.
7. Shau D, Patton R, Patel S, et al., 2018, Synthetic mesh vs. Allograft extensor mechanism reconstruction in total knee arthroplasty – A systematic review of the literature and meta– analysis. Knee, 25(1): 2.
8. Wang Z, Wang C, Li C, et al., 2017, Analysis of factors influencing bone ingrowth into three–dimensional printed porous metal scaffolds: A review. J Alloys Compd, 717: 271–285.
9. Kumar A, Mandal S, Barui S, et al., 2016, Low temperature additive manufacturing of three dimensional scaffolds for bone–tissue engineering applications: Processing related challenges and property assessment. Mater Sci Eng R, 103: 1–39.
10. Chevalier E, Chulia D, Pouget C, et al., 2008, Fabrication of porous substrates: A review of processes using pore forming agents in the biomaterial field. J Pharm Sci, 97(3): 1135–1154.
11. Pia G, Casnedi L, Ionta M, et al., 2015, On the elastic deformation properties of porous ceramic materials obtained by pore–forming agent method. Ceram Int, 41(9): 11097–11105.
12. Moghadam M Z, Hassanajili S, Esmaeilzadeh F, et al., 2017, Formation of porous HPCL/LPCL/HA scaffolds with supercritical CO2 gas foaming method. J Mech Behav Biomed Mater, 69: 115.
13. Costantini M, Colosi C, Mozetic P, et al., 2016, Correlation between porous texture and cell seeding efficiency of gas foaming and microfluidic foaming scaffolds. Mater Sci Eng C Mater Biol Appl, 62: 668–677.
14. Theodorou G S, Eleana K, Anna T, et al., 2016, Sol–Gel derived Mg–based ceramic scaffolds doped with zinc or copper ions: Preliminary results on their synthesis, characterization, and biocompatibility. Int J Biomater, 2016(1–2): 3858301.
15. Ros–Tárraga P, Murciano A, Mazón P, et al., 2017, New 3D stratified Si–Ca–P porous scaffolds obtained by sol–gel and polymer replica method: Microstructural, mineralogical and chemical characterization. Ceram Int, 43(8): 6548–6553.
16. Abdkhorsand S, Sabersamandari S, 2017, Development of nanocomposite scaffolds based on TiO2 doped in grafted chitosan/hydroxyapatite by freeze drying method and evaluation of biocompatibility. Int J Biol Macromol, 101: 51–58.
17. Fereshteh Z, Fathi M, Bagri A, et al., 2016, Preparation and characterization of aligned porous PCL/zein scaffolds as drug delivery systems via improved unidirectional freeze–drying method. Mater Sci Eng C Mater Biol Appl, 68: 613–622.
18. Janik H M, 2015, A review: Fabrication of porous polyurethane scaffolds. Mater Sci Eng C Mater Biol Appl, 48: 586.
19. Bose S, Ke D, Sahasrabudhe H, et al., 2017, Additive manufacturing of biomaterials. Prog Mater Sci, 93:1-310.
20. Parthasarathy J, 2014, 3D modeling, custom implants and its future perspectives in craniofacial surgery. Ann Maxillofac Surg, 4(1): 9.
21. Jardini A L, Larosa M A, Bernardes L F, et al., 2014, Customised titanium implant fabricated in additive manufacturing for craniomaxillofacial surgery. Virtual Phys Prototyp, 9(2): 115–125.
22. Avaliable from: http://www.csiro.au/en/News/News–releases/ 2014/3D–Heel–In–World–First–Surgery2014.
23. Song W, Chen L, Seta J, et al., 2017, Corona discharge: A novel approach to fabricate three–dimensional electrospun nanofibers. Acs Biomater Sci Eng, 3(6): 1146–1153.
24. Hollister S J, Flanagan C L, Morrison R J, et al., 2016, Integrating image–based design and 3D biomaterial printing to create patient specific devices within a design control framework for clinical translation. Acs Biomater Sci Eng, 2(10): 1658-1661.
25. Shuai C, Guo W, Gao C, et al., 2018, An nMgO containing scaffold: Antibacterial activity, degradation properties and cell responses. Int J Bioprint, 4(1): 34-578.
26. He J, Xu F, Dong R, et al., 2017, Electrohydrodynamic 3D printing of microscale poly (ε–caprolactone) scaffolds with multi–walled carbon nanotubes. Biofabrication, 9(1): 15007.
27. Guvendiren M, Molde J, Soares R, et al., 2016, Designing biomaterials for 3D printing. Acs Biomater Sci Eng, 2(10): 1679–1693.
28. Calignano F, 2014, Design optimization of supports for overhanging structures in aluminum and titanium alloys by selective laser melting. Mater Design, 64(9): 203–213.
29. Yan R, Luo D, Huang H, et al., 2018, Electron beam melting in the fabrication of three–dimensional mesh titanium mandibular prosthesis scaffold. Sci Rep, 8(1): 750.
30. Yu G Z, Chou D T, Hong D, et al., 2017, Biomimetic rotated lamellar plywood motifs by additive manufacturing of metal alloy scaffolds for bone tissue engineering. Acs Biomater Sci Eng, 3(4): 649–657.
31. Feng J, Fu J, Shang C, et al., 2018, Porous scaffold design by solid T–splines and triply periodic minimal surfaces. Comput Methods Appl Mech Eng, 336, 333–352.
32. Probst F A, Hutmacher D W, Müller D F, et al., 2010, Calvarial reconstruction by customized bioactive implant. Handchir Mikrochir Plast Chir, 42(6): 369.
33. Naing M W, Chua C K, Leong K F, et al., 2005, Fabrication of customised scaffolds using computer-aided design and rapid prototyping techniques. Rapid Prototyp J, 11(4): 249– 259.
34. Ovsianikov A, Deiwick A, Vlierberghe S V, et al., 2011, Laser fabrication of three–dimensional CAD scaffolds from photosensitive gelatin for applications in tissue engineering. Biomacromolecules, 12(4): 851–858.
35. Yu K M, Chiu W K, Yeung Y C, 2006, Toolpath generation for layer manufacturing of fractal objects. Rapid Prototyp J, 12(4): 214–221.
36. Sing S L, Wiria F Eand Yeong W Y, 2018, Selective laser melting of lattice structures: A statistical approach to manufacturability and mechanical behavior. Robot Comput Integr Manuf, 49: 170–180.
37. Duan B, Cheung W, Land W M, 2011, Optimized fabrication of Ca–P/PHBV nanocomposite scaffolds via selective laser sintering for bone tissue engineering. Biofabrication, 3(1): 15001.
38. Melchels F P, Bertoldi K, Gabbrielli R, et al., 2010, Mathematically defined tissue engineering scaffold architectures prepared by stereolithography. Biomaterials, 31(27): 6909.
39. Sercombe T B, Xu X, Challis V J, et al., 2015, Failure modes in high strength and stiffness to weight scaffolds produced by selective laser melting. Mater Des, 67: 501–508.
40. Murr L E, Gaytan S M, Medina F, et al., 2010, Next– generation biomedical implants using additive manufacturing of complex, cellular and functional mesh arrays. Philos Trans, 368(1917): 1999.
41. Cheah C M, Chua C K, Leong K F, et al., 2004, Automatic algorithm for generating complex polyhedral scaffold structures for tissue engineering. Tissue Eng, 10(4): 595–610.
42. Lu G, Xu S, Yan Q S, et al., 2013, Study on dimension change law from CAD model to prototype of rapid investment casting based on selective laser sintering. Adv Mater Res, 774–776(774–776): 1046–1050.
43. Florczyk S J, Simon M, Juba D, et al., 2017, A bioinformatics 3D cellular morphotyping strategy for assessing biomaterial scaffold niches. Acs Biomater Sci Eng, 3(10): 2302-2313.
44. Kerativitayanan P, Tatullo M, Khariton M, et al., 2017, Nanoengineered osteoinductive and elastomeric scaffolds for bone tissue engineering. Acs Biomater Sci Eng, 3(4): 590–600.
45. Thomas R C, Vu P, Shan P M, et al., 2017, Sacrificial crystal templated hyaluronic acid hydrogels as biomimetic 3D tissue scaffolds for nerve tissue regeneration. Acs Biomater Sci Eng, 3(7): 1172–1174.
46. Altamimi A A, Fernandes P R A, Peach C, et al., 2017, Metallic bone fixation implants: A novel design approach for reducing the stress shielding phenomenon. Virtual Phys Prototyp, 12(2): 141–151.
47. Osanov J K, 2016, Topology optimization for architected materials design. Ann Rev Mater Res, 46(1): 211–233.
48. Guest J Kand Prévost J H, 2007, Design of maximum permeability material structures. Comput Methods Appl Mech Eng, 196(4–6): 1006–1017.
49. Guest J, Kand P J, 2006, Optimizing multifunctional materials: Design of microstructures for maximized stiffness and fluid permeability. Int J Solids Struct, 43(22): 7028–7047.
50. Sturm S, Zhou S, Mai Y W, et al., 2010, On stiffness of scaffolds for bone tissue engineering – A numerical study. J Biomech, 43(9): 1738–1744.
51. Huang, X X, 2010, Evolutionary Topology Optimization of Continuum Structures: Methods and Applications. Hoboken, New Jersey: Wiley.
52. Huang X, Xie Y M, 2011, Topological design of microstructures of cellular materials for maximum bulk or shear modulus. Comput Mater Sci, 50(6): 1861–1870.
53. Osher S, Sethian J A, 1988, Fronts propagating with curvature–dependent speed: Algorithms based on Hamilton– Jacobi formulations. J Comput Phys, 79(1): 12–49.
54. Li Q Z, 2008, A Variational Level set Method for the Topology Optimization of Steady–State Navier–Stokes Flow. USA: Academic Press Professional, Inc.
55. Challis V, Guest J K, 2010, Level set topology optimization of fluids in stokes flow. Int J Num Methods Eng, 79(10): 1284–1308.
56. Takezawa A, Kobashi M, Takezawa A, et al., 2017, Design methodology for porous composites with tunable thermal expansion produced by multi–material topology optimization and additive manufacturing. Compos Part B Eng, 131: 1-283.
57. Hollister S J, Levy R A, Chu T M, et al., 2000, An image– based approach for designing and manufacturing craniofacial scaffolds. Int J Oral Maxillofac Surg, 29(1): 67–71.
58. Giannitelli S M, Accoto D, Trombetta M, et al., 2014, Current trends in the design of scaffolds for computer – Aided tissue engineering. Acta Biomater, 10(2): 580–594.
59. Sun W, Starly B, Nam J, et al., 2005, Bio–CAD modeling and its applications in computer–aided tissue engineering. Comput Aided Des, 37(11): 1097–1114.
60. Hollister S J, Chu T M, Guldberg R E, et al., 2002, Image Based Design and Manufacture of Scaffolds for Bone Reconstruction. IUTAM Symposium on Synthesis in Bio Solid Mechanics. pp163-174.
61. Hollister S J, 2005, Porous scaffold design for tissue engineering. Nat Mater, 4(7): 518–524.
62. Podshivalov L, Gomes C M, Zocca A, et al., 2013, Design, analysis and additive manufacturing of porous structures for biocompatible micro–scale scaffolds -. Procedia Cirp, 5(1): 247–252.
63. Meyer U, Runte C, Dirksen D, et al., 2003, Image–Based Biomimetric Approach to Design and Fabrication of Tissue Engineered Bone. Cars 2003. Computer Assisted Radiology and Surgery. Proceedings of the International Congress and Exhibition, London. pp726–732.
64. Pattanayak D K, Fukuda A, Matsushita T, et al., 2011, Bioactive ti metal analogous to human cancellous bone: Fabrication by selective laser melting and chemical treatments. Acta Biomater, 7(3): 1398–1406.
65. Terasaki O, 2005, Band structure of the P, D, and G surfaces. Phys Rev B, 72(8):085459.
66. Lai M, Kulak A N, Law D, et al., 2007, Profiting from nature: macroporous copper with superior mechanical properties. Chem Commun, 34(34): 3547–3549.
67. Robb R A, 2005, Schwarz Meets Schwann: Design and Fabrication of Biomorphic and Durataxic Tissue Engineering Scaffolds. Palm Springs, CA, USA: Medical Image Computing and Computer–assisted Intervention–miccai, International Conference. pp794–801.
68. Kapfer S C, Hyde S T, Mecke K, et al., 2011, Minimal surface scaffold designs for tissue engineering. Biomaterials, 32(29): 6875–6882.
69. Melchels F W, Barradas A M, Blitterswijk C A, et al., 2010, Effects of the architecture of tissue engineering scaffolds on cell seeding and culturing. Acta Biomater, 6(11): 4208–4217.
70. Yang N, Quan Z, Zhang D, et al., 2014, Multi–morphology transition hybridization CAD design of minimal surface porous structures for use in tissue engineering. Comput Aided Des, 56(11): 11–21.
71. Yang N, Wang S, Gao L, et al., 2017, Building implicit– surface–based composite porous architectures. Compos Struct, 173: 35–43.
72. Zhou K Y, 2014, Effective method for multi–scale gradient
porous scaffold design and fabrication. Mater Sci Eng C Mater Biol Appl, 43: 502–505.
73. Yang N, Zhang D T, 2015, Novel real function based method to construct heterogeneous porous scaffolds and additive manufacturing for use in medical engineering. Med Eng Phys, 37(11): 1037–1046.
74. Yang N, Du C F, Wang S, et al., 2016, Mathematically defined gradient porous materials. Mater Lett, 173: 136–140.
75. Yoo D J, 2011, Porous scaffold design using the distance field and triply periodic minimal surface models. Biomaterials, 32(31): 7741.
76. Yoo D J, 2013, Heterogeneous porous scaffold design using the continuous transformations of triply periodic minimal surface models. Int J Precis Eng Manuf, 14(10): 1743–1753.
77. Yoo D, Kim K H, 2015, An advanced multi–morphology porous scaffold design method using volumetric distance field and beta growth function. Int J Precis Eng Manuf, 16(9): 2021–2032.
78. Yang N, Zhou K G, 2015, Simple method to generate and fabricate stochastic porous scaffolds. Mater Sci Eng C, 56: 444–450.
79. Roberts A, Garboczi E J, 2001, Elastic moduli of model random three–dimensional closed–cell cellular solids. Acta Mater, 49(2): 189–197.
80. Okabe A, Boots B, Sugihara K, et al., 2001, Spatial Tessellations: Concepts and Applications of Voronoi Diagrams. New York, NY: John Wiley & Sons, Inc.
81. Kou X, Tan S T, 2010, A simple and effective geometric representation for irregular porous structure modeling. Comput Aided Des, 42(10): 930–941.
82. Kou X Y, Tan S T, 2012, Microstructural modelling of functionally graded materials using stochastic voronoi diagram and B–Spline representations. Int J Comput Integr Manuf, 25(2): 177–188.
83. Chow H N, Tan S T, Sze W S, 2007, Layered modeling of porous structures with voronoi diagrams. Comput Aided Des Appl, 4(1–4): 321–330.
84. Fantini M, Curto M, Crescenzio F D, 2016, A method to design biomimetic scaffolds for bone tissue engineering based on voronoi lattices. Virtual Phys Prototyp, 11(2): 77–90.
85. Curto M F, 2017, Interactive design and manufacturing of a voronoi–based biomimetic bone scaffold for morphological characterization. Int J Interact Des Manuf, 6: 1–12.
86. Gómez S, Vlad M D, López J, et al., 2016, Design and properties of 3D scaffolds for bone tissue engineering. Acta Biomater, 42: 341–350.
87. Wang G, Shen L, Zhao J, et al., 2018, Design and compressive behavior of controllable irregular porous scaffolds: Based on voronoi–tessellation and for additive manufacturing. Acs Biomater Sci Eng, 4(2): 719–727.
88. Tumbleston J R, Shirvanyants D, Ermoshkin N, et al., 2015, Continuous liquid interface production of 3D objects. Science, 347(6228): 1349–1352.
89. Xing J–F, Zheng M–Land Duan X–M, 2015, Two–photon polymerization microfabrication of hydrogels: an advanced 3D printing technology for tissue engineering and drug delivery. Chemical Society Reviews, 44(15): 5031–5039.
90. Yu T, Richards D J, Trusk T C, et al., 2014, 3D Printing facilitated scaffold–free tissue unit fabrication. Biofabrication, 6(2): 24111.
91. Pourchet L J, Thepot A, Albouy M, et al., 2016, Human skin 3D bioprinting using scaffold–free approach. Adv Healthc Mater, 6(4): 1601101.
92. Cervera G B, Lombera G, 1999, Numerical prediction of temperature and density distributions in selective laser sintering processes. Rapid Prototyp J, 5(1): 21–26.
93. Gu D C, 2016, Effect of metallurgical defect and phase transition on geometric accuracy and wear resistance of iron– based parts fabricated by selective laser melting. J Mater Res, 31(10): 1477–1490.
94. Senatov F, Niaza K, Zadorozhnyy M Y, et al., 2016, Mechanical properties and shape memory effect of 3D– printed PLA–based porous scaffolds. J Mech Behav Biomed Mater, 57: 139–148.
95. Jezierski A, Rennie K, Zurakowski B, et al., 2014, Neuroprotective effects of GDNF–expressing human amniotic fluid cells. Stem Cell Rev Rep, 10(2): 251–268.
96. Shuai C, Li Y, Feng P, et al., 2018, Positive feedback effects of Mg on the hydrolysis of poly–l–lactic acid (PLLA): Promoted degradation of PLLA scaffolds. Polym Test, 68: 27–33.
97. Yang L, Li J, Jin Y, et al., 2015, In vitro enzymatic degradation of the cross–linked poly (ε–caprolactone) implants. Polym Degrad Stab, 112: 10–19.
98. Du Y, Liu H, Yang Q, et al., 2017, Selective laser sintering scaffold with hierarchical architecture and gradient composition for osteochondral repair in rabbits. Biomaterials, 137: 37.
99. Du Y, Liu H, Shuang J, et al., 2015, Microsphere–based selective laser sintering for building macroporous bone scaffolds with controlled microstructure and excellent biocompatibility. Colloids Surf B Biointerfaces, 135: 81.
100. Kumaresan T, Gandhinathan R, Ramu M, et al., 2016, Design, analysis and fabrication of polyamide/hydroxyapatite porous structured scaffold using selective laser sintering method for bio–medical applications. J Mech Sci Technol, 30(11): 5305–5312.
101. Shuai C, Gao C, Nie Y, et al., 2011, Structure and properties of nano–hydroxypatite scaffolds for bone tissue engineering with a selective laser sintering system. Nanotechnology, 22(28): 285703.
102. Shuai C, Li P, Liu J, et al., 2013, Optimization of TCP/HAP ratio for better properties of calcium phosphate scaffold via selective laser sintering. Mater Charact, 77(3): 23–31.
103. Liu J, Hu H, Li P, et al., 2013, Fabrication and characterization of porous 45S5 glass scaffolds via direct selective laser sintering. Mater Manuf Process, 28(6): 610–615.
104. Sing S L, Yeong W Y, Wiria F E, et al., 2017, Direct selective laser sintering and melting of ceramics: A review. Rapid Prototyp J, 23(3): 611–623.
105. Liu J, Gao C, Feng P, et al., 2015, A bioactive glass nanocomposite scaffold toughed by multi–wall carbon nanotubes for tissue engineering. J Ceram Soc Jpn, 123(1438): 485–491.
106. Gao C, Pei F, Peng S, et al., 2017, Carbon nanotubes, graphene and boron nitride nanotubes reinforced bioactive ceramics for bone repair. Acta Biomater, 61: 1.
107. Järvenpää A, Karjalainen P, Mäntyjärvi K, 2012, Passive laser assisted bending of ultra–high strength steels. Adv Mater Res, 418–420: 1542–1547.
108. Gao C, Liu T, Shuai C, et al., 2014, Enhancement mechanisms of graphene in nano–58S bioactive glass scaffold: Mechanical and biological performance. Sci Rep, 4(4): 4712.
109. Duan S, Feng P, Gao C, et al., 2015, Microstructure evolution and mechanical properties improvement in liquid–phase– sintered hydroxyapatite by laser sintering. Materials, 8(3): 1162–1175.
110. Liu D, Zhuang J, Shuai C, et al., 2013, Mechanical properties’ improvement of a tricalcium phosphate scaffold with poly–l– lactic acid in selective laser sintering. Biofabrication, 5(2): 25005.
111. Gu D, Hagedorn Y C, Meiners W, et al., 2012, Densification behavior, microstructure evolution, and wear performance of selective laser melting processed commercially pure titanium. Acta Mater, 60(9): 3849–3860.
112. Čapek J, Machová M, Fousová M, et al., 2016, Highly porous, low elastic modulus 316L stainless steel scaffold prepared by selective laser melting. Mater Sci Eng C, 69: 631–639.
113. Weißmann V, Bader R, Hansmann H, et al., 2016, Influence of the structural orientation on the mechanical properties of selective laser melted Ti6Al4V open–porous scaffolds. Mater Des, 95: 188–197.
114. Wang L, Kang J, Sun C, et al., 2017, Mapping porous microstructures to yield desired mechanical properties for application in 3D printed bone scaffolds and orthopaedic implants. Mater Des, 133: 62–68.
115. Shah F A, Snis A, Matic A, et al., 2016, 3D printed Ti6Al4V implant surface promotes bone maturation and retains a higher density of less aged osteocytes at the bone–implant interface. Acta Biomater, 30: 357–367.
116. Yang Y, Guo X, He C, et al., 2018, Regulating degradation behavior by incorporating mesoporous silica for Mg bone implants. Acs Biomater Sci Eng, 4(3): 1046–1054.
117. Deng Y, Yang Y, Gao C, et al., 2018, Mechanism for corrosion protection of β–TCP reinforced ZK60 via laser rapid solidification. Int J Bioprint, 4(1): 124.
118. Shuai C, Xue L, Gao C, et al., Selective laser melting of Zn–Ag alloys for bone repair: Microstructure, mechanical properties and degradation behaviour. Virtual Phys Prototyp, 13(3): 1-9.
119. Yang Y, Yuan F, Gao C, et al., 2018, A combined strategy to enhance the properties of Zn by laser rapid solidification and laser alloying. J Mech Behav Biomed Mater, 82: 51–60.
120. Sing S L, An J, Yeong W Y, et al., 2016, Laser and electron– beam powder–bed additive manufacturing of metallic implants: A review on processes, materials and designs. J Orthop Res, 34(3): 369–385.
121. Shuai C, Yang Y, Wu P, et al., 2017, Laser rapid solidification improves corrosion behavior of Mg–Zn–Zr alloy. J Alloys Comp, 691: 961–969.
122. Shuai C, He C, Feng P, et al., 2017, Biodegradation mechanisms of selective laser–melted Mg–xAl–Zn alloy: Grain size and intermetallic phase. Virtual Phys Prototyp, 13(2): 1–11.
123. Yang Y, Wu P, Lin X, et al., 2016, System development, formability quality and microstructure evolution of selective laser–melted magnesium. Virtual Phys Prototyp, 11(3): 173–181.
124. Li Y, Zhou J, Pavanram P, et al., 2018, Additively manufactured biodegradable porous magnesium. Acta Biomater, 67: 378–392.
125. Grasso M, Demir A, Previtali B, et al., 2018, In situ monitoring of selective laser melting of zinc powder via infrared imaging of the process plume. Robot Comput Integr Manuf, 49: 229–239.
126. Wen P, Jauer L, Voshage M, et al., 2018, Densification behavior of pure Zn metal parts produced by selective laser melting for manufacturing biodegradable implants. J Mater Process Technol, 258: 128–137.
127. Demir A G, Monguzzi L, Previtali B, 2017, Selective laser melting of pure Zn with high density for biodegradable implant manufacturing. Add Manuf, 15: 20–28.
128. Montani M, Demir A G, Mostaed E, et al., 2017, Processability of pure Zn and pure Fe by SLM for biodegradable metallic implant manufacturing. Rapid Prototyp J, 23(3): 514–523.
129. Hou Y, Jia G, Yue R, et al., 2018, Synthesis of biodegradable Zn–based scaffolds using NaCl templates: Relationship between porosity, compressive properties and degradation behavior. Mater Charact, 137: 305–315.
130. Hutmacher D W, 2000, Scaffolds in tissue engineering bone and cartilage. Biomaterials, 21(24): 2529–2543.
131. Zhou C, Yang K, Wang K, et al., 2016, Combination of fused deposition modeling and gas foaming technique to fabricated hierarchical macro/microporous polymer scaffolds. Mater Des, 109: 415–424.
132. Tellis B C, Szivek J A, Bliss C L, et al., 2008, Trabecular scaffolds created using micro CT guided fused deposition modeling. Mater Sci Eng C, 28(1): 171–178.
133. Kosorn W, Sakulsumbat M, Uppanan P, et al., 2017, PCL/ PHBV blended three dimensional scaffolds fabricated by fused deposition modeling and responses of chondrocytes to the scaffolds. J Biomed Mater Res Part B Appl Biomater, 105(5): 1141.
134. De S R, D’Amora U, Russo T, et al., 2015, 3D fibre deposition and stereolithography techniques for the design of multifunctional nanocomposite magnetic scaffolds. J Mater Sci Mater Med, 26(10): 250.
135. Vaezi M, Yang S, 2015, Extrusion–based additive manufacturing of PEEK for biomedical applications. Virtual Phys Prototyp, 10(3): 123–135.
136. Rinaldi M, Ghidini T, Cecchini F, et al., 2018, Additive layer manufacturing of poly (ether ether ketone) via FDM. Compos Part B Eng, 145: 162-172.
137. Shim J H, Won J Y, Sung S J, et al., 2015, Comparative efficacies of a 3D–printed PCL/PLGA/β–TCP membrane and a titanium membrane for guided bone regeneration in beagle dogs. Polymers, 7(10): 2061–2077.
138. Youssef A, Hollister S J, Dalton P D, 2017, Additive manufacturing of polymer melts for implantable medical devices and scaffolds. Biofabrication, 9(1): 12002.
139. Xu N, Ye X, Wei D, et al., 2014, 3D artificial bones for bone repair prepared by computed tomography–guided fused deposition modeling for bone repair. Acs Appl Mater Interfaces, 6(17): 14952–14963.
140. Kim J, Mcbride S, Tellis B, et al., 2012, Rapid–prototyped PLGA/β–TCP/hydroxyapatite nanocomposite scaffolds in a rabbit femoral defect model. Biofabrication, 4(2): 25003.
141. Poh P S, Hutmacher D W, Holzapfel B M, et al., 2016, In vitro and in vivo bone formation potential of surface calcium phosphate–coated polycaprolactone and polycaprolactone/ bioactive glass composite scaffolds. Acta Biomater, 30: 319–333.
142. Frazier W E, 2014, Metal additive manufacturing: A review. J Mater Eng Perform, 23(6): 1917–1928.
143. Liang Hand Harris R, 2008, Customised Implants for Bone Replacement and Growth. US: Springer.
144. Ataee A, Li Y, Fraser D, et al., 2018, Anisotropic Ti–6Al–4V gyroid scaffolds manufactured by electron beam melting (EBM) for bone implant applications. Mater Des, 137: 1-480.
145. Surmeneva M, Surmenev R, Chudinova E, et al., 2017, Fabrication of multiple–layered gradient cellular metal scaffold via electron beam melting for segmental bone reconstruction. Mater Des, 133: 195-204.
146. Li S J, Xu Q S, Wang Z, et al., 2014, Influence of cell shape on mechanical properties of Ti–6Al–4V meshes fabricated by electron beam melting method. Acta Biomater, 10(10): 4537–4547.
147. Shah F A, Omar O, Suska F, et al., 2016, Long–term osseointegration of 3D printed CoCr constructs with an interconnected open–pore architecture prepared by electron beam melting. Acta Biomater, 36: 296–309.
148. Zhao S, Li S J, Hou W T, et al., 2016, The influence of cell morphology on the compressive fatigue behavior of Ti–6Al– 4V meshes fabricated by electron beam melting. J Mech Behav Biomed Mater, 59: 251–264.
149. Zhao B, Wang H, Qiao N, et al., 2016, Corrosion resistance characteristics of a Ti–6Al–4V alloy scaffold that is fabricated by electron beam melting and selective laser melting for implantation in vivo. Mater Sci Eng C, 70(Pt 1): 832–841.
150. Lv J, Jia Z, Li J, et al., 2015, Electron beam melting fabrication of porous Ti6Al4V scaffolds: Cytocompatibility and osteogenesis. Adv Eng Mater, 17(9): 1391–1398.
151. Algardh J K, Horn T, West H, et al., 2016, Thickness dependency of mechanical properties for thin–walled titanium parts manufactured by electron beam melting (EBM) ®;∗. Add Manuf, 12: 45–50.
152. Eldesouky I, Harrysson O, West H, et al., 2017, Electron beam melted scaffolds for orthopedic applications. Add Manuf, 17: 169-175.
153. Rännar L E, Gustafson C Gand Glad A, 2008, Efficient cooling with tool inserts manufactured by electron beam melting. Rapid Prototyp J, 13(3): 128–135.
154. Palaganas N, Mangadlao J, De A L, et al., 2017, 3D printing of photocurable cellulose nanocrystal composite for fabrication of complex architectures via stereolithography. Acs Appl Mater Interfaces, 9(39): 34314–34324.
155. Wan Q, Tian J, Liu M, et al., 2015, Surface modification of carbon nanotubes via combination of mussel inspired chemistry and chain transfer free radical polymerization. Appl Surf Sci, 346: 335–341.
156. Li B, Hou W, Sun J, et al., 2015, Tunable functionalization of graphene oxide sheets through surface–initiated cationic polymerization. Macromolecules, 48(4): 994–1001.
157. Elomaa L, Teixeira S, Hakala R, et al., 2011, Preparation of poly(ε–caprolactone)–based tissue engineering scaffolds by stereolithography. Acta Biomater, 7(11): 3850–3856.
158. Hockaday L A, Kang K H, Colangelo N W, et al., 2012, Rapid 3D printing of anatomically accurate and mechanically heterogeneous aortic valve hydrogel scaffolds. Biofabrication, 4(3): 35005.
159. Meyer W, Engelhardt S, Novosel E, et al., 2012, Soft polymers for building up small and smallest blood supplying systems by stereolithography. J Funct Biomater, 3(2): 257–268.
160. Guillaume O, Geven M A, Sprecher C M, et al., 2017, Surface–enrichment with hydroxyapatite nanoparticles in stereolithography–fabricated composite polymer scaffolds promotes bone repair. Acta Biomater, 54: 386-398.
161. Thavornyutikarn B, Tesavibul P, Sitthiseripratip K, et al., 2017, Porous 45S5 bioglass®–based scaffolds using stereolithography: Effect of partial pre–sintering on structural and mechanical properties of scaffolds. Mater Sci Eng C Mater Biol Appl, 75: 1281.
162. Du D, Asaoka T, Ushida T, et al., 2014, Fabrication and perfusion culture of anatomically shaped artificial bone using stereolithography. Biofabrication, 6(4): 45002.
163. Levy R A, Chu T M, Halloran J W, et al., 1997, CT–generated porous hydroxyapatite orbital floor prosthesis as a prototype bioimplant. Ajnr Am J Neuroradiol, 18(8): 1522–1525.
164. Sabree I, Gough J E, Derby B, 2015, Mechanical properties of porous ceramic scaffolds: Influence of internal dimensions. Ceram Int, 41(7): 8425–8432.
165. Kim J Y, Jin W L, Lee S J, et al., 2007, Development of a bone scaffold using HA nanopowder and micro–stereolithography technology. Microelectronic Eng, 84(5–8): 1762–1765.
166. Melchels F P, Feijen J, Grijpma D W, 2010, A review on stereolithography and its applications in biomedical engineering. Biomaterials, 31(24): 6121–6130.
167. Sun B, Long Y Z, Zhang H D, et al., 2014, Advances in three– dimensional nanofibrous macrostructures via electrospinning. Prog Polym Sci, 39(5): 862–890.
168. Hochleitner G, JãNgst T, Brown T D, et al., 2015, Additive manufacturing of scaffolds with sub–micron filaments via melt electrospinning writing. Biofabrication, 7(3): 35002.
169. Tian L, Prabhakaran M P, Hu J, et al., 2016, Synergistic effect of topography, surface chemistry and conductivity of the electrospun nanofibrous scaffold on cellular response of PC12 cells. Colloids Surf B Biointerfaces, 145: 420–429.
170. Wang P, Wang Y, Tong L, 2013, Functionalized polymer nanofibers: A versatile platform for manipulating light at the nanoscale. Light Sci Appl, 2(10): e102.
171. Repanas A, Andriopoulou S, Glasmacher B, 2016, The significance of electrospinning as a method to create fibrous scaffolds for biomedical engineering and drug delivery applications. J Drug Deliv Sci Technol, 31: 137–146.
172. Cipitria A, 2011, Design, fabrication and characterization of PCL electrospun scaffolds - A review. J Mater Chem, 21(26): 9419–9453.
173. Luu Y K, Kim K, Hsiao B S, et al., 2003, Development of a nanostructured DNA delivery scaffold via electrospinning of PLGA and PLA–PEG block copolymers. J Controll Release, 89(2): 341–353.
174. Brown J H, Das P, Divito M D, et al., 2018, Nanofibrous PLGA electrospun scaffolds modified with Type I collagen influence hepatocyte function and support viability in vitro. Acta Biomater, 73: 217–227.
175. Valente T A M, Silva D M, Gomes P S, et al., 2016, Effect of sterilization methods on electrospun poly (lactic acid) (PLA) fiber alignment for biomedical applications. Acs Appl Mater Interfaces, 8(5): 3241.
176. Shim I K, Mi R J, Kim K H, et al., 2010, Novel three-dimensional scaffolds of poly (L-lactic acid) microfibers using electrospinning and mechanical expansion: Fabrication and bone regeneration. J Biomed Mater Res Part B Appl Biomater, 95B(1): 150–160.
177. Vaquette C, Ivanovski S, Hamlet S M, et al., 2013, Effect of culture conditions and calcium phosphate coating on ectopic bone formation. Biomaterials, 34(22): 5538–5551.
178. Yao Q, Cosme J G, Xu T, et al., 2016, Three dimensional electrospun PCL/PLA blend nanofibrous scaffolds with significantly improved stem cells osteogenic differentiation and cranial bone formation. Biomaterials, 115: 115.
179. Tan R P, Chan A, Lennartsson K, et al., 2018, Integration of induced pluripotent stem cell–derived endothelial cells with polycaprolactone/gelatin–based electrospun scaffolds for enhanced therapeutic angiogenesis. Stem Cell Res Ther, 9(1): 70.
180. Li K, Sun H, Sui H, et al., 2015, Composite mesoporous silica nanoparticle/chitosan nanofibers for bone tissue engineering. Rsc Adv, 5(23): 17541–17549.
181. Yong, D, 2015, In vitro and in vivo evaluation of the developed PLGA/HAp/Zein scaffolds for bone–cartilage interface regeneration. Biomedical and Environmental Sciences, 28(1): 1.
182. Bagchi A, Meka S R, Rao B N, et al., 2014, Perovskite ceramic nanoparticles in polymer composites for augmenting bone tissue regeneration. Nanotechnology, 25(48): 485101.
183. Rezvani Z, Venugopal J R, Urbanska A M, et al., 2016, A bird’s eye view on the use of electrospun nanofibrous scaffolds for bone tissue engineering: Current state–of–the– art, emerging directions and future trends. Nanomedicine, 12(7): 2181–2200.
184. Min S K, Kim J H, Singh R K, et al., 2015, Therapeutic– designed electrospun bone scaffolds: Mesoporous bioactive nanocarriers in hollow fiber composites to sequentially deliver dual growth factors. Acta Biomater, 16(1): 103–116.
185. Rogina A, 2014, Electrospinning process: Versatile preparation method for biodegradable and natural polymers and biocomposite systems applied in tissue engineering and drug delivery. Appl Surf Sci, 296(8): 221–230.
186. Kenawy E, Abdelhay F I, Elnewehy M H, et al., 2015, Processing of polymer nanofibers through electrospinning as drug delivery systems. Mater ChemPhys, 113(1): 296–302.
187. Kolambkar Y M, Dupont K M, Boerckel J D, et al., 2011, An alginate–based hybrid system for growth factor delivery in the functional repair of large bone defects. Biomaterials, 32(1): 65.
188. Aleni A H, Ituarte I F, Mohite A, et al., 2017, Comparing stiffness of solid and scaffold nano–TiO 2 structures produced by material extrusion method. Ceram Int, 44(2): 2231-2239.
189. Huang W, Zhang X, Wu Q, et al., 2013, Fabrication of HA/β- TCP scaffolds based on micro-syringe extrusion system. Rapid Prototyp J, 19(5): 319–326.
190. Zhou K, Dong C, Zhang X, et al., 2015, Preparation and characterization of nanosilver–doped porous hydroxyapatite scaffolds. Ceram Int, 41(1): 1671–1676.
191. Chen Z, Zhang X, Yang Y, et al., 2017, Fabrication and characterisation of 3D complex hydroxyapatite scaffolds with hierarchical porosity of different features for optimal bioactive performance. Ceram Int, 43(1): 336–344.
192. Feng P, Niu M, Gao C, et al., 2014, A novel two–step sintering for nano–hydroxyapatite scaffolds for bone tissue engineering. Sci Rep, 4: 5599.
193. Liu F H, 2014, Synthesis of biomedical composite scaffolds by laser sintering: Mechanical properties and in vitro bioactivity evaluation. Appl Surf Sci, 297(297): 1–8.
194. Thöne M, Leuders S, Riemer A, et al., 2012, Influence of Heat–Treatment on Selective Laser Melting Products – e.g. Ti6Al4V. Austin: Annual International Solid Freeform Fabrication Symposium.
195. Wauthle R, Vrancken B, Beynaerts B, et al., 2015, Effects of build orientation and heat treatment on the microstructure and mechanical properties of selective laser melted Ti6Al4V lattice structures. Add Manuf, 5: 77–84.
196. Zhao X, Lui Y S, Choo C K, et al., 2015, Calcium phosphate coated keratin–PCL scaffolds for potential bone tissue regeneration. Mater Sci Eng C, 49: 746–753.
197. Luo Y, Lode A, Wu C, et al., 2015, Alginate/nanohydroxyapatite scaffolds with designed core/shell structures fabricated by 3D plotting and in situ mineralization for bone tissue engineering. Acs Appl Mater Interfaces, 7(12): 6541–6549.
198. Rifai A, Tran N, Dwm L, et al., 2018, Polycrystalline diamond coating of additively manufactured titanium for biomedical applications. Acs Appl Mater Interfaces, 10(10): 8474–8484.
199. Chen H, Wang C, Xiao Y, et al., 2016, Construction of surface HA/TiO 2 coating on porous titanium scaffolds and its preliminary biological evaluation. Mater Sci Eng C Mater Biol Appl, 70(Pt 2): 1047.
200. Chai Y C, Kerckhofs G, Roberts S J, et al., 2012, Ectopic bone formation by 3D porous calcium phosphate–Ti6Al4V hybrids produced by perfusion electrodeposition. Biomaterials, 33(16): 4044–4058.
201. Liao H T, Lee M Y, Tsai W W, et al., 2013, Osteogenesis of adipose-derived stem cells on polycaprolactone–β-tricalcium phosphate scaffold fabricated via selective laser sintering and surface coating with collagen Type I. J Tissue Eng Regen Med, 10(10): E337.
202. Chen C H, Lee M Y, Shyu V B, et al., 2014, Surface modification of polycaprolactone scaffolds fabricated via selective laser sintering for cartilage tissue engineering. Mater Sci Eng C, 40: 389–397.
203. Huang W, Zhang H, Huang Y, et al., 2011, Hierarchical porous carbon obtained from animal bone and evaluation in electric double–layer capacitors. Carbon, 49(3): 838–843.
204. Amin Y S, Van d S J, Chai Y C, et al., 2014, Bone regeneration performance of surface–treated porous titanium. Biomaterials, 35(24): 6172–6181.
205. Cheng A, Humayun A, Cohen D J, et al., 2014, Additively manufactured 3D porous Ti–6Al–4V constructs mimic trabecular bone structure and regulate osteoblast proliferation, differentiation and local factor production in a porosity and surface roughness dependent manner. Biofabrication, 6(4): 45007.
206. Shuai C, Yang Y, Feng P, et al., 2018, A multi–scale porous scaffold fabricated by a combined additive manufacturing and chemical etching process. Int J Bioprint, 4(3): 133.
207. Ramier J, Boubaker M B, Guerrouache M, et al., 2014, Novel routes to epoxy functionalization of PHA-based electrospun scaffolds as ways to improve cell adhesion. J Polym Sci Part A Polym Chem, 52(6): 816–824.
208. Wang X, Li Y, Hodgson P D, et al., 2010, Biomimetic modification of porous TiNbZr alloy scaffold for bone tissue engineering. Tissue Eng Part A, 16(1): 309–316.
209. Hanson A D, Wall M E, Pourdeyhimi B, et al., 2007, Effects of oxygen plasma treatment on adipose–derived human mesenchymal stem cell adherence to poly (L–lactic acid) scaffolds. J Biomater Sci Polym Ed, 18(11): 1387–1400.
210. Roh H S, Lee C M, Hwang Y H, et al., 2016, Addition of MgO nanoparticles and plasma surface treatment of three– dimensional printed polycaprolactone/hydroxyapatite scaffolds for improving bone regeneration. Mater Sci Eng C., 74: 1-608.
211. Roh H S, Jung S C, Kook M S, et al., 2016, In vitro study of 3D PLGA/n–HAp/β–TCP composite scaffolds with etched oxygen plasma surface modification in bone tissue engineering. Appl Surf Sci, 388: 321–330.
212. Jeon H, Lee H, Kim G, 2014, A surface–modified poly(ɛ–caprolactone) scaffold comprising variable nanosized surface–roughness using a plasma treatment. Tissue Eng Part C Methods, 20(12): 951.
213. Jayanth N, Senthil P, Prakash C, 2018, Effect of chemical treatment on tensile strength and surface roughness of 3D– printed ABS using the FDM process. Virtual Phys Prototyp, 2018: 1–9.
214. Calignano F, 2018, Investigation of the accuracy and roughness in the laser powder bed fusion process. Virtual Phys Prototyp, 1: 1–8.