Bioprinting of tumor immune microenvironment for immunotherapy
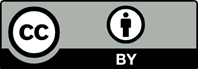
Accurately simulating the tumor immune microenvironment (TIME), which consists of a tumor, extracellular matrix (ECM), vascular network, and a variety of stromal and immune cells, is crucial for advancing and testing immunotherapies such as checkpoint inhibitors, chimeric antigen receptor-T (CAR-T) cells, and cancer vaccines. Traditional models, such as animal models, are limited by their differences from human immune environments. Bioprinting addresses these limitations by incorporating tumors, immune cells, and vascular cells within an ECM, thereby reflecting the complex interactions, including trafficking, between cancer and immune cells. These models provide better predictive accuracy for human immune responses, reducing translational failures and improving preclinical testing. While bioprinting methods for simulating the tumor microenvironment, where cancer cells form spheroids surrounded by blood vessels, are well reviewed, bioprinting methods for recapitulating the TIME are not as thoroughly explored. This review aims to fill this gap by exploring the development, application, and potential of bioprinted TIME models in enhancing the study and efficacy of immunotherapies, ultimately offering a more realistic and personalized approach to cancer treatment.

- Yaddanapudi K, Mitchell RA, Eaton JW. Cancer vaccines: looking to the future. Oncoimmunology. 2013;2(3):e23403. doi: 10.4161/onci.23403
- Flores-Torres S, Jiang T, Kort-Mascort J, et al. Constructing 3D in vitro models of heterocellular solid tumors and stromal tissues using extrusion-based bioprinting. ACS Biomater Sci Eng. 2023;9(2):542-561. doi: 10.1021/acsbiomaterials.2c00998
- Zhou Z, Pang Y, Ji J, et al. Harnessing 3D in vitro systems to model immune responses to solid tumours: a step towards improving and creating personalized immunotherapies. Nat Rev Immunol. 2024;24(1):18-32. doi: 10.1038/s41577-023-00896-4
- Zhang Z, Chen X, Gao S, et al. 3D bioprinted tumor model: a prompt and convenient platform for overcoming immunotherapy resistance by recapitulating the tumor microenvironment. Cell Oncol. 2024;47:1113-1126. doi: 10.1007/s13402-024-00935-9
- Staros R, Michalak A, Rusinek K, et al. Perspectives for 3D-bioprinting in modeling of tumor immune evasion. Cancers. 2022;14(13):3126. doi: 10.3390/cancers14133126
- Parodi I, Di Lisa D, Pastorino L, et al. 3D bioprinting as a powerful technique for recreating the tumor microenvironment. Gels. 2023;9(6):482. doi: 10.3390/gels9060482
- Voskoglou-Nomikos T, Pater JL, Seymour L. Clinical predictive value of the cell line, human xenograft, and mouse allograft preclinical cancer models. Clin Cancer Res. 2003;9(11):4227-4239.
- González-Callejo P, García-Astrain C, Herrero-Ruiz A, et al. 3D bioprinted tumor-stroma models of triple-negative breast cancer stem cells for preclinical targeted therapy evaluation. ACS Appl Mater Interfaces. 2024;16(21):27151-27163. doi: 10.1021/acsami.4c04135
- Augustine R, Kalva SN, Ahmad R, et al. 3D bioprinted cancer models: revolutionizing personalized cancer therapy. Transl Oncol. 2021;14(4):101015. doi: 10.1016/j.tranon.2021.101015
- Zhu W, Holmes B, Glazer RI, Zhang LG. 3D printed nanocomposite matrix for the study of breast cancer bone metastasis. Nanomed Nanotechnol Biol Med. 2016; 12(1):69-79. doi: 10.1016/j.nano.2015.09.010
- Bae J, Han S, Park S. Recent advances in 3D bioprinted tumor microenvironment. Biochip J. 2020;14:137-147. doi: 10.1007/s13206-020-4201-8
- Zhang YS, Duchamp M, Oklu R, et al. Bioprinting the cancer microenvironment. ACS Biomater Sci Eng. 2016;2(10): 1710-1721. doi: 10.1021/acsbiomaterials.6b00246
- Shukla P, Yeleswarapu S, Heinrich MA, et al. Mimicking tumor microenvironment by 3D bioprinting: 3D cancer modeling. Biofabrication. 2022;14(3):Article 032002. doi: 10.1088/1758-5090/ac6d11
- Monestime S, Lazaridis D. Pexidartinib (TURALIO): the first FDA-indicated systemic treatment for tenosynovial giant cell tumor. Drugs R D. 2020;20(3):189-195. doi: 10.1007/s40268-020-00314-3
- Pardoll DM. The blockade of immune checkpoints in cancer immunotherapy. Nat Rev Cancer. 2012;12(4):252-264. doi: 10.1038/nrc3239
- Topalian SL, Drake CG, Pardoll DM. Immune checkpoint blockade: a common denominator approach to cancer therapy. Cancer Cell. 2015;27(4):450-461. doi: 10.1016/j.ccell.2015.03.001
- June CH, O’Connor RS, Kawalekar OU, et al. CAR T cell immunotherapy for human cancer. Science. 2018;359(6382):1361-1365. doi: 10.1126/science.aar6711
- Gross G, Waks T, Eshhar Z. Expression of immunoglobulin- T-cell receptor chimeric molecules as functional receptors with antibody-type specificity. Proc Natl Acad Sci U S A. 1989;86(24):10024-10028. doi: 10.1073/pnas.86.24.10024
- Schlom J. Therapeutic cancer vaccines: current status and moving forward. J Natl Cancer Inst. 2012;104(8):599-613. doi: 10.1093/jnci/djs033
- Finn OJ. Cancer vaccines: between the idea and the reality. Nat Rev Immunol. 2003;3(8):630-641. doi: 10.1038/nri1150
- Mou P, Ge QH, Sheng R, et al. Research progress on the immune microenvironment and immunotherapy in gastric cancer. Front Immunol. 2023;14:1291117. doi: 10.3389/fimmu.2023.1291117
- Chen C, Liu X, Chang CY, et al. The interplay between T cells and cancer: the basis of immunotherapy. Genes. 2023;14(5):1008. doi: 10.3390/genes14051008
- Cullen SP, Brunet M, Martin SJ. Granzymes in cancer and immunity. Cell Death Differ. 2010;17(4):616-623. doi: 10.1038/cdd.2009.206
- Navasardyan I, Bonavida B. Regulation of T cells in cancer by nitric oxide. Cells. 2021;10(10):2655. doi: 10.3390/cells10102655
- McDonnell AM, Robinson BW, Currie AJ. Tumor antigen cross‐presentation and the dendritic cell: where it all begins? J Immunol Res. 2010;2010(1):539519. doi: 10.1155/2010/539519
- Chen Y, Song Y, Du W, et al. Tumor-associated macrophages: an accomplice in solid tumor progression. J Biomed Sci. 2019;26(1):78. doi: 10.1186/s12929-019-0568-z
- Yu Y. The function of NK cells in tumor metastasis and NK cell-based immunotherapy. Cancers. 2023;15(8):2323. doi: 10.3390/cancers15082323
- Gabrilovich D. Mechanisms and functional significance of tumour-induced dendritic-cell defects. Nat Rev Immunol. 2004;4(12):941-952. doi: 10.1038/nri1498
- Melief CJ. Cancer immunotherapy by dendritic cells. Immunity. 2008;29(3):372-383. doi: 10.1016/j.immuni.2008.08.004
- Shurin GV, Ma Y, Shurin MR. Immunosuppressive mechanisms of regulatory dendritic cells in cancer. Cancer Microenviron. 2013;6(2):159-167. doi: 10.1007/s12307-013-0133-3
- Cess CG, Finley SD. Multi-scale modeling of macrophage—T cell interactions within the tumor microenvironment. PLoS Comp Biol. 2020;16(12):e1008519. doi: 10.1371/journal.pcbi.1008519
- Ito H, Seishima M. Regulation of the induction and function of cytotoxic T lymphocytes by natural killer T cell. J Biomed Biotechnol. 2010;2010:641757. doi: 10.1155/2010/641757
- Zanna MY, Yasmin A, Omar AR, et al. Review of dendritic cells, their role in clinical immunology, and distribution in various animal species. Int J Mol Sci. 2021; 22(15):8044. doi: 10.3390/ijms22158044
- Xiao Z, Wang R, Wang X, et al. Impaired function of dendritic cells within the tumor microenvironment. Front Immunol. 2023;14:1213629. doi: 10.3389/fimmu.2023.1213629
- Lendeckel U, Venz S, Wolke C. Macrophages: shapes and functions. ChemTexts. 2022;8(2):12. doi: 10.1007/s40828-022-00163-4
- Vivier E, Tomasello E, Baratin M, et al. Functions of natural killer cells. Nat Immunol. 2008;9(5):503-510. doi: 10.1038/ni1582
- Mu P, Zhou S, Lv T, et al. Newly developed 3D in vitro models to study tumor–immune interaction. J Exp Clin Cancer Res. 2023;42(1):81. doi: 10.1186/s13046-023-02653-w
- Zschaler J, Schlorke D, Arnhold J. Differences in innate immune response between man and mouse. Crit Rev Immunol. 2014;34(5):433-454. doi: 10.1615/CritRevImmunol.2014011600
- Bjornson-Hooper ZB, Fragiadakis GK, Spitzer MH, et al. A comprehensive atlas of immunological differences between humans, mice, and non-human primates. Front Immunol. 2022;11(13):867015. doi: 10.3389/fimmu.2022.867015
- Zhao Z, Zhang S, Jiang N, et al. Patient-derived immunocompetent tumor organoids: a platform for chemotherapy evaluation in the context of T-cell recognition. Angew Chem Int Ed. 2024;63(9): e202317613. doi: 10.1002/anie.202317613
- Goldenson BH, Hor P, Kaufman DS. iPSC-derived natural killer cell therapies-expansion and targeting. Front Immunol. 2022;3(13):841107. doi: 10.3389/fimmu.2022.841107
- Minagawa A, Yoshikawa T, Yasukawa M, et al. Enhancing T cell receptor stability in rejuvenated iPSC-derived T cells improves their use in cancer immunotherapy. Cell Stem Cell. 2018;23(6):850-858.e4. doi: 10.1016/j.stem.2018.10.005
- Li Y, Hermanson DL, Moriarity BS, Kaufman DS. Human iPSC-derived natural killer cells engineered with chimeric antigen receptors enhance anti-tumor activity. Cell Stem Cell. 2018;23(2):181-192.e5. doi: 10.1016/j.stem.2018.06.002
- Zhou Y, Li M, Zhou K, et al. Engineering induced pluripotent stem cells for cancer immunotherapy. Cancers. 2022;14(9):2266. doi: 10.3390/cancers14092266
- Abraham RT, Weiss A. Jurkat T cells and development of the T-cell receptor signalling paradigm. Nat Rev Immunol. 2004;4(4):301-308. doi: 10.1038/nri1330
- Klingemann H, Boissel L, Toneguzzo F. Natural killer cells for immunotherapy–advantages of the NK-92 cell line over blood NK cells. Front Immunol. 2016; 14(7):91. doi: 10.3389/fimmu.2016.00091
- Chanput W, Mes JJ, Wichers HJ. THP-1 cell line: an in vitro cell model for immune modulation approach. Int Immunopharmacol. 2014;23(1):37-45. doi: 10.1016/j.intimp.2014.08.002
- Gong J-H, Maki G, Klingemann HG. Characterization of a human cell line (NK-92) with phenotypical and functional characteristics of activated natural killer cells. Leukemia. 1994;8(4):652-658.
- Laskowski TJ, Biederstadt A, Rezvani K. Natural killer cells in antitumour adoptive cell immunotherapy. Nat Rev Cancer. 2022;22(10):557-575. doi: 10.1038/s41568-022-00491-0
- Zhu H, Blum RH, Bjordahl R, et al. Pluripotent stem cell-derived NK cells with high-affinity noncleavable CD16a mediate improved antitumor activity. Blood. 2020;135(6):399-410. doi: 10.1182/blood.2019000621
- Lu P, Weaver VM, Werb Z. The extracellular matrix: a dynamic niche in cancer progression. J Cell Biol. 2012;196(4):395-406. doi: 10.1083/jcb.201102147
- Mai Z, Lin Y, Lin P, et al. Modulating extracellular matrix stiffness: a strategic approach to boost cancer immunotherapy. Cell Death Dis. 2024;15(5):307. doi: 10.1038/s41419-024-06697-4
- Park Y, Lee D, Lee JE, et al. The matrix stiffness coordinates the cell proliferation and PD-L1 expression via YAP in lung adenocarcinoma. Cancers. 2024;16(3):598. doi: 10.3390/cancers16030598
- Hynes RO. The extracellular matrix: not just pretty fibrils. Science. 2009;326(5957):1216-1219. doi: 10.1126/science.1176009
- Joyce JA, Fearon DT. T cell exclusion, immune privilege, and the tumor microenvironment. Science. 2015; 348(6230):74-80. doi: 10.1126/science.aaa6204
- Madsen CD, Sahai E. Cancer dissemination—lessons from leukocytes. Dev Cell. 2010;19(1):13-26. doi: 10.1016/j.devcel.2010.06.013
- Noy R, Pollard JW. Tumor-associated macrophages: from mechanisms to therapy. Immunity. 2014;41(1):49-61. doi: 10.1016/j.immuni.2014.06.010
- Lee KY, Mooney DJ. Alginate: properties and biomedical applications. Prog Polym Sci. 2012;37(1):106-126. doi: 10.1016/j.progpolymsci.2011.06.003
- Yang D, Jones KS. Effect of alginate on innate immune activation of macrophages. J Biomed Mater Res. 2009;90(2):411-418. doi: 10.1002/jbm.a.32096
- Alipal J, Pu’Ad NM, Lee T, et al. A review of gelatin: properties, sources, process, applications, and commercialisation. Mater Today: Proc. 2021;42(1):240-250. doi: 10.1016/j.matpr.2020.12.922
- Elalouf A. Immune response against the biomaterials used in 3D bioprinting of organs. Transpl Immunol. 2021;69:101446. doi: 10.1016/j.trim.2021.101446
- Necas J, Bartosikova L, Brauner P, Kolar J. Hyaluronic acid (hyaluronan): a review. Vet Med. 2008;53(8):397-411. doi: 10.17221/1930-VETMED
- Haas A. The phagosome: compartment with a license to kill. Traffic. 2007;8(4):311-330. doi: 10.1111/j.1600-0854.2006.00531.x
- Johnson P, Ruffell B. CD44 and its role in inflammation and inflammatory diseases. Inflamm Allergy Drug Targets. 2009;8(3):208-220. doi: 10.2174/187152809788680994
- Assmann V, Fieber C, Herrlich P, et al. CD44 is the principal mediator of hyaluronic-acid-induced melanoma cell proliferation. J Invest Dermatol. 2001;116(1):93-101. doi: 10.1046/j.1523-1747.2001.00236.x
- Avila Rodríguez MI, Rodríguez Barroso LG, Sánchez ML. Collagen: a review on its sources and potential cosmetic applications. J Cosmet Dermatol. 2018;17(1):20-26. doi: 10.1111/jocd.12450
- Lynn A, Yannas I, Bonfield W. Antigenicity and immunogenicity of collagen. J Biomed Mater Res B Appl Biomater. 2004;71(2):343-354. doi: 10.1002/jbm.b.30096
- Kuczek DE, Larsen AMH, Thorseth M-L, et al. Collagen density regulates the activity of tumor-infiltrating T cells. J Immunother Cancer. 2019;7(1):68. doi: 10.1186/s40425-019-0556-6
- Kou SG, Peters LM, Mucalo MR. Chitosan: a review of sources and preparation methods. Int J Biol Macromol. 2021;169:85-94. doi: 10.1016/j.ijbiomac.2020.12.005
- Oliveira MI, Santos SG, Oliveira MJ, et al. Chitosan drives anti-inflammatory macrophage polarisation and pro-inflammatory dendritic cell stimulation. Eur Cell Mater. 2012;24(136):133-136. doi: 10.22203/ecm.v024a10
- Almeida CR, Serra T, Oliveira MI, et al. Impact of 3-D printed PLA-and chitosan-based scaffolds on human monocyte/macrophage responses: unraveling the effect of 3-D structures on inflammation. Acta Biomater. 2014;10(2): 613-622. doi: 10.1016/j.actbio.2013.10.035
- Kasravi M, Ahmadi A, Babajani A, et al. Immunogenicity of decellularized extracellular matrix scaffolds: a bottleneck in tissue engineering and regenerative medicine. Biomater Res. 2023;27(1):10. doi: 10.1186/s40824-023-00348-z
- Yue K, Trujillo-de Santiago G, Alvarez MM, et al. Synthesis, properties, and biomedical applications of gelatin methacryloyl (GelMA) hydrogels. Biomaterials. 2015;73:254-271. doi: 10.1016/j.biomaterials.2015.08.045
- Donaldson AR, Tanase CE, Awuah D, et al. Photocrosslinkable gelatin hydrogels modulate the production of the major pro-inflammatory cytokine, TNF-α, by human mononuclear cells. Front Bioeng Biotechnol. 2018;6:116. doi: 10.3389/fbioe.2018.00116
- Chakraborty J, Roy S, Ghosh S. Regulation of decellularized matrix mediated immune response. Biomater Sci. 2020;8(5):1194-1215. doi: 10.1039/c9bm01780a
- Heinrich MA, Bansal R, Lammers T, et al. 3D‐bioprinted mini‐brain: a glioblastoma model to study cellular interactions and therapeutics. Adv Mater. 2019;31(14):1806590. doi: 10.1002/adma.201806590
- Reynolds DS, de Lázaro I, Blache ML, et al. Microporogen-structured collagen matrices for embedded bioprinting of tumor models for immuno-oncology. Adv Mater. 2023;35(33):e2210748. doi: 10.1002/adma.202210748
- Morley CD, Flores CT, Drake JA, et al. Spatiotemporal T cell dynamics in a 3D bioprinted immunotherapy model. Bioprinting. 2022;28:e00231. doi: 10.1016/j.bprint.2022.e00231
- Mazzaglia C, Sheng Y, Rodrigues LN, et al. Deployable extrusion bioprinting of compartmental tumoroids with cancer associated fibroblasts for immune cell interactions. Biofabrication. 2023;15(2):025005. doi: 10.1088/1758-5090/acb1db
- Flores-Torres S, Dimitriou NM, Pardo LA, et al. Bioprinted multicomponent hydrogel co-culture tumor-immune model for assessing and simulating tumor-infiltrated lymphocyte migration and functional activation. ACS Appl Mater Interfaces. 2023;15(28):33250-33262. doi: 10.1021/acsami.3c02995
- Datta P, Dey M, Ataie Z, et al. 3D bioprinting for reconstituting the cancer microenvironment. NPJ Precis Oncol. 2020;4(1):18. doi: 10.1038/s41698-020-0121-2
- Langhans SA. Three-dimensional in vitro cell culture models in drug discovery and drug repositioning. Front Pharmacol. 2018;9:6. doi: 10.3389/fphar.2018.00006
- Jenkins RW, Barbie DA, Flaherty KT. Mechanisms of resistance to immune checkpoint inhibitors. Br J Cancer. 2018;118(1):9-16. doi: 10.1038/bjc.2017.434
- Cattaneo CM, Dijkstra KK, Fanchi LF, et al. Tumor organoid-T-cell coculture systems. Nat Protoc. 2020;15(1): 15-39. doi: 10.1038/s41596-019-0232-9
- Jin Z, Li X, Zhang X, et al. Engineering the fate and function of human T-Cells via 3D bioprinting. Biofabrication. 2021;13(3):035016. doi: 10.1088/1758-5090/abd56b
- Kim D, Jo S, Lee D, et al. NK cells encapsulated in micro/ macropore-forming hydrogels via 3D bioprinting for tumor immunotherapy. Biomater Res. 2023;27(1):60. doi: 10.1186/s40824-023-00403-9
- Xu Y, Zhu W, Wu J, et al. 3D‐printed dendritic cell vaccines for post‐surgery cancer immunotherapy. Adv Funct Mater. 2024;34(33):2400507. doi: 10.1002/adfm.202400507
- Li C, Jin B, Sun H, et al. Exploring the function of stromal cells in cholangiocarcinoma by three-dimensional bioprinting immune microenvironment model. Front Immunol. 2022;13:941289. doi: 10.3389/fimmu.2022.941289
- Grolman JM, Zhang D, Smith AM, et al. Rapid 3D extrusion of synthetic tumor microenvironments. Adv Mater. 2015;27(37):5512. doi: 10.1002/adma.201501729
- Gong Z, Huang L, Tang X, et al. Acoustic droplet printing tumor organoids for modeling bladder tumor immune microenvironment within a week. Adv Healthc Mater. 2021;10(22):2101312. doi: 10.1002/adhm.202101312
- Xu Y, Saiding Q, Zhou X, et al. Electrospun fiber‐based immune engineering in regenerative medicine. Smart Med. 2024;3(1):e20230034. doi: 10.1002/SMMD.20230034
- Tang M, Xie Q, Gimple RC, et al. Three-dimensional bioprinted glioblastoma microenvironments model cellular dependencies and immune interactions. Cell Res. 2020;30(10):833-853. doi: 10.1038/s41422-020-0338-1
- Dey M, Kim MH, Nagamine M, et al. Biofabrication of 3D breast cancer models for dissecting the cytotoxic response of human T cells expressing engineered MAIT cell receptors. Biofabrication. 2022;14(4):044105. doi: 10.1088/1758-5090/ac925a
- Ayan B, Heo DN, Zhang Z, et al. Aspiration-assisted bioprinting for precise positioning of biologics. Sci Adv. 2020;6(10):eaaw5111. doi: 10.1126/sciadv.aaw5111
- Cao X, Ashfaq R, Cheng F, et al. A tumor‐on‐a‐chip system with bioprinted blood and lymphatic vessel pair. Adv Funct Mater. 2019;29(31):1807173. doi: 10.1002/adfm.201807173
- Dey M, Kim MH, Dogan M, et al. Chemotherapeutics and CAR‐T cell‐based immunotherapeutics screening on a 3D bioprinted vascularized breast tumor model. Adv Funct Mater. 2022;32(52):2203966. doi: 10.1002/adfm.202203966
- Kim JH, Lee S, Kang SJ, et al. Establishment of three-dimensional bioprinted bladder cancer-on-a-chip with a microfluidic system using Bacillus Calmette–Guérin. Int J Mol Sci. 2021;22(16):8887. doi: 10.3390/ijms22168887
- Goodarzi Hosseinabadi H, Dogan E, Miri AK, Ionov L. Digital light processing bioprinting advances for microtissue models. ACS Biomater Sci Eng. 2022;8(4):1381-1395. doi: 10.1021/acsbiomaterials.1c01509
- El-Gamal MI, Al-Ameen SK, Al-Koumi DM, et al. Recent advances of colony-stimulating factor-1 receptor (CSF- 1R) kinase and its inhibitors. J Med Chem. 2018;61(13): 5450-5466. doi: 10.1021/acs.jmedchem.7b00873
- Binnemars‐Postma K, Bansal R, Storm G, Prakash J. Targeting the Stat6 pathway in tumor‐associated macrophages reduces tumor growth and metastatic niche formation in breast cancer. FASEB J. 2018;32(2): 969-978. doi: 10.1096/fj.201700629R
- Wang W, Wang X, Yang W, et al. A CTLA-4 blocking strategy based on Nanoboby in dendritic cell-stimulated cytokine-induced killer cells enhances their anti-tumor effects. BMC Cancer. 2021;21(1):1029. doi: 10.1186/s12885-021-08732-5
- Terren I, Orrantia A, Vitalle J, et al. NK cell metabolism and tumor microenvironment. Front Immunol. 2019;10:2278. doi: 10.3389/fimmu.2019.02278
- Kankeu Fonkoua LA, Sirpilla O, Sakemura R, et al. CAR T cell therapy and the tumor microenvironment: current challenges and opportunities. Mol Ther Oncolytics. 2022;25:69-77. doi: 10.1016/j.omto.2022.03.009
- Grunewald L, Lam T, Andersch L, et al. A reproducible bioprinted 3D tumor model serves as a preselection tool for CAR T cell therapy optimization. Front Immunol. 2021;12:689697. doi: 10.3389/fimmu.2021.689697
- Nguyen DT, Ogando-Rivas E, Liu R, et al. CAR T cell locomotion in solid tumor microenvironment. Cells. 2022;11(12):1974. doi: 10.3390/cells11121974
- Morse MA, Gwin III WR, Mitchell DA. Vaccine therapies for cancer: then and now. Target Oncol. 2021;16(2):121-152. doi: 10.1007/s11523-020-00788-w
- Langer EM, Allen-Petersen BL, King SM, et al. Modeling tumor phenotypes in vitro with three-dimensional bioprinting. Cell Rep. 2019;26(3):608-623. e6. doi: 10.1016/j.celrep.2018.12.090
- Huang Y, Wang S, Guo Q, et al. Optical coherence tomography detects necrotic regions and volumetrically quantifies multicellular tumor spheroids. Cancer Res. 2017;77(21):6011-6020. doi: 10.1158/0008-5472.CAN-17-0821
- Park J, Park B, Kim TY, et al. Quadruple ultrasound, photoacoustic, optical coherence, and fluorescence fusion imaging with a transparent ultrasound transducer. Proc Natl Acad Sci U S A. 2021;118(11):e1920879118. doi: 10.1073/pnas.1920879118
- Gao Q, Yang L, Lu M, et al. The artificial intelligence and machine learning in lung cancer immunotherapy. J Hematol Oncol. 2023;16(1):55. doi: 10.1186/s13045-023-01456-y